Transporters and Drug-Metabolizing Enzymes in Drug Toxicity
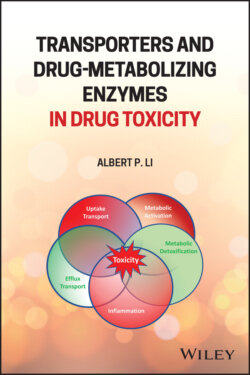
Реклама. ООО «ЛитРес», ИНН: 7719571260.
Оглавление
Albert P. Li. Transporters and Drug-Metabolizing Enzymes in Drug Toxicity
Table of Contents
List of Tables
List of Illustrations
Guide
Pages
Transporters and Drug‐Metabolizing Enzymes in Drug Toxicity
Preface
List of Contributors
1 Overview: Drug Metabolism, Transporter‐Mediated Uptake and Efflux, and Drug Toxicity
1.1 Drug Toxicity as a Challenge in Drug Development
1.2 Fate of an Orally Administered Drug
1.3 The Multiple Determinant Hypothesis for Idiosyncratic Drug Toxicity
1.4 Concluding Remarks
1.4.1 A Comprehensive Approach to Safety Evaluation in Drug Development
1.4.2 The Dose Makes the Poison – Paracelsus Updated
References
2 Transporter, Drug Metabolism, and Drug‐Induced Liver Injury in Marketed Drugs
2.1 Introduction
2.2 Hepatic Metabolism
2.2.1 Phase I Metabolism
2.2.2 Phase II Metabolism
2.3 Reactive Metabolite Formation and Assessment
2.3.1 Metabolism and Reactive Metabolites
2.3.2 Dose and Reactive Mtabolites
2.3.3 Structural Alerts for Avoiding Reactive Metabolites
2.3.4 Experimental Approaches for Assessing Reactive Metabolites
2.3.4.1 Covalent Binding Assay
2.3.4.2 Electrophile Trapping Experiments
2.3.4.3 Time Dependent Inactivation of CYP450 Enzymes
2.4 Hepatic Transporters
2.5 Genetic Variants and Their Impact for Pharmacokinetic Behavior and Safety
2.5.1 CYP3A4
2.5.2 CYP3A5
2.5.3 CYP2D6
2.5.4 CYP2C9
2.5.5 CYP2C19
2.5.6 CYP2B6
2.5.7 UGT1A1
2.5.8 NAT2
2.5.9 Hepatic Transporters
2.6 Summary
Acknowledgment
Disclaimer
References
3 Drug‐Metabolism Enzymes and Transporter Activities as Risk Factors of Selected Marketed Drugs Associated with Drug‐Induced Fatalities
3.1 Introduction
3.2 Acetaminophen
3.2.1 Drug Metabolism and Toxicity
3.2.2 Transporters and Toxicity
3.2.3 Risk Factors
3.3 Cerivastatin
3.3.1 Drug Metabolism and Toxicity
3.3.2 Transporter and Toxicity
3.3.3 Risk Factors
3.4 Felbamate
3.4.1 Drug Metabolism and Toxicity
3.4.2 Transporters and Toxicity
3.4.3 Risk Factors
3.5 Flucloxacillin
3.5.1 Drug Metabolism and Toxicity
3.5.2 Transporters and Toxicity
3.5.3 Risk Factors
3.6 Nefazodone
3.6.1 Drug Metabolism and Toxicity
3.6.2 Transporters and Toxicity
3.6.3 Risk Factors
3.7 Obeticholic Acid
3.7.1 Drug Metabolism and Toxicity
3.7.2 Transporters and Toxicity
3.7.3 Risk Factors
3.8 Sitaxentan
3.8.1 Drug Metabolism and Toxicity
3.8.2 Transporters and Toxicity
3.8.3 Risk Factors
3.9 Sorivudine
3.9.1 Drug Metabolism and Toxicity
3.9.2 Transporters and Toxicity
3.9.3 Risk Factors
3.10 Tacrine
3.10.1 Drug Metabolism and Toxicity
3.10.2 Transporters and Toxicity
3.10.3 Risk Factors
3.11 Terfenadine
3.11.1 Drug Metabolism and Toxicity
3.11.2 Transporter and Toxicity
3.11.3 Risk Factors
3.12 Troglitazone (Rezulin®)
3.12.1 Drug Metabolism and Toxicity
3.12.2 Transporter and Toxicity
3.12.3 Risk Factors
3.13 Trovafloxacin
3.13.1 Metabolism and Toxicity
3.13.2 Transporters and Toxicity
3.13.3 Risk Factors
3.14 Conclusions
References
4 Drug‐Metabolizing Enzymes and Drug Toxicity
4.1 Introduction
4.2 Drug‐Metabolism Enzymes Involved in Metabolic Activation and Detoxification
4.3 Cytochrome P450 Monooxygenase (CYP)
4.3.1 CYP1A
4.3.1.1 Metabolic Activation
4.3.1.2 Drug Substrates
4.3.1.3 Inducers
4.3.1.4 Inhibitors
4.3.1.5 Individual Variations
4.3.1.6 Involvement in Drug Toxicity
4.3.2 CYP2A6
4.3.2.1 Substrates
4.3.2.2 Inducers
4.3.2.3 Inhibitors
4.3.2.4 Individual Variations
4.3.2.5 Involvement in Drug Toxicity
4.3.3 CYP2B6
4.3.3.1 Substrates
4.3.3.2 Inducers
4.3.3.3 Inhibitors
4.3.3.4 Individual Variations
4.3.3.5 Involvement in Drug Toxicity
4.3.4 CYP2C8
4.3.4.1 Substrates
4.3.4.2 Inducers
4.3.4.3 Inhibitors
4.3.4.4 Individual Variations
4.3.4.5 Involvement in Drug Toxicity
4.3.5 CYP2C9
4.3.5.1 Substrates
4.3.5.2 Inducers
4.3.5.3 Inhibitors
4.3.5.4 Individual Variations
4.3.5.5 Involvement in Drug Toxicity
4.3.6 CYP2C19
4.3.6.1 Substrates
4.3.6.2 Inducers
4.3.6.3 Inhibitors
4.3.6.4 Individual Variations
4.3.6.5 Involvement in Drug Toxicity
4.3.7 CYP2D6
4.3.7.1 Substrates
4.3.7.2 Inducers
4.3.7.3 Inhibitors
4.3.7.4 Individual Variations
4.3.7.5 Involvement in Drug Toxicity
4.3.8 CYP2E1
4.3.8.1 Substrates
4.3.8.2 Inducers
4.3.8.3 Inhibitors
4.3.8.4 Involvement in Drug Toxicity
4.3.9 CYP2J2
4.3.9.1 Substrates
4.3.9.2 Inhibitors
4.3.9.3 Inducers
4.3.9.4 Individual Variations
4.3.9.5 Involvement in Drug Toxicity
4.3.10 CYP3A
4.3.10.1 Substrates
4.3.10.2 Inducers
4.3.10.3 Inhibitors
4.3.10.4 Individual Variations
4.3.10.5 Involvement in Drug Toxicity
4.4 Non‐P450 Drug‐Metabolizing Enzymes. 4.4.1 Flavin‐Containing Monooxygenases (FMOs)
4.4.1.1 Substrates
4.4.1.2 Inducers
4.4.1.3 Inhibitors
4.4.1.4 Individual Variations
4.4.1.5 Involvement in Drug Toxicity
4.4.2 Monoamine Oxidase (MAO)
4.4.2.1 Substrates
4.4.2.2 Inducers
4.4.2.3 Inhibitors
4.4.2.4 Individual Variations
4.4.2.5 Involvement in Drug Toxicity
4.4.3 Alcohol Dehydrogenase (ADH) and Aldehyde Dehydrogenase (ALDH)
4.4.3.1 Substrates
4.4.3.2 Inducers
4.4.3.3 Inhibitors
4.4.3.4 Individual Variations
4.4.3.5 Involvement in Drug Toxicity
4.4.4 Aldehyde Oxidase (AOX)
4.4.4.1 Substrates
4.4.4.2 Inducers
4.4.4.3 Inhibitors
4.4.4.4 Individual Variations
4.4.4.5 Involvement in drug toxicity
4.4.5 Carboxylesterases (CESs)
4.4.5.1 Substrates
4.4.5.2 Inducers
4.4.5.3 Inhibitors
4.4.5.4 Individual Variations
4.4.5.5 Involvement in Drug Toxicity
4.4.6 N‐Acetyltransferase (NAT)
4.4.6.1 Substrates
4.4.6.2 Inducers
4.4.6.3 Inhibitors
4.4.6.4 Individual Variations
4.4.6.5 Involvement in Drug Toxicity
4.4.7 Glutathione Transferase (GST)
4.4.7.1 Substrates
4.4.7.2 Inducers
4.4.7.3 Inhibitors
4.4.7.4 Individual Variations
4.4.7.5 Involvement in Drug Toxicity
4.4.8 Methyltransferase (MT)
4.4.8.1 Substrates
4.4.8.2 Inhibitors
4.4.8.3 Individual Variations
4.4.8.4 Involvement in Drug Toxicity
4.4.9 Uridine Glucuronosyltransferase (UGT)
4.4.9.1 Substrates
4.4.9.2 Inducers
4.4.9.3 Inhibitors
4.4.9.4 Individual Variations
4.4.9.5 Involvement in Drug Toxicity
4.4.10 Sulfotransferase (SULT)
4.4.10.1 Substrates
4.4.10.2 Inducers
4.4.10.3 Inhibitors
4.4.10.4 Individual Variations
4.4.10.5 Involvement in Drug Toxicity
4.5 Conclusions
References
5 Genetic Polymorphism of Drug‐Metabolizing Enzymes and Drug Transporters in Drug Toxicity
5.1 Introduction
5.2 Drug‐Induced Liver Injury. 5.2.1 Background
5.2.2 Polymorphisms Affecting Drug Metabolism and DILI
5.2.2.1 Isoniazid
5.2.2.2 Diclofenac
5.2.2.3 Tolcapone
5.2.2.4 Ticlopidine
5.2.2.5 Efavirenz
5.2.2.6 Troglitazone
5.2.3 Polymorphisms Affecting Transporters and DILI
5.3 Drug‐Induced Skin Injury and Related Hypersensitivity Reactions
5.4 Statin‐Induced Myopathy. 5.4.1 Background
5.4.2 Cytochromes P450
5.4.3 Transporters
5.5 Conclusions
References
6 Acyl Glucuronidation and Acyl‐CoA Formation Mechanisms Mediating the Bioactivation and Potential Toxicity of Carboxylic Acid‐containing Drugs
6.1 Introduction
6.2 Phase II Metabolism. 6.2.1 Glucuronidation
6.2.2 Acyl‐CoA Thioester Formation
6.3 Chemical Stability of Phase II Metabolites. 6.3.1 Acyl Glucuronide Instability
6.3.2 Acyl‐CoA Thioester Stability
6.4 Phase II Metabolite Chemical Reactivity. 6.4.1 Acyl Glucuronide Reactivity with Nucleophiles In vitro
6.4.2 Acyl‐CoA Thioester Reactivity with Nucleophiles In vitro
6.5 Phase II Metabolite‐Mediated Covalent Binding. 6.5.1 Acyl Glucuronide‐Mediated Covalent Binding to Protein
6.5.2 Acyl‐CoA Thioester‐Mediated Covalent Binding to Protein
6.6 Phase II Metabolite Prediction of Covalent Binding. 6.6.1 Prediction of Covalent Binding to Protein by Acyl Glucuronides
6.6.2 Prediction of Covalent Binding to Protein by Acyl‐CoA Thioesters
6.7 Studies Directly Comparing Carboxylic Acid Drug Bioactivation by Acyl Glucuronidation and Acyl‐CoA Formation
6.8 Prediction of Drug‐Induced Liver Injury for Carboxylic Acid Drugs
6.9 Conclusions
References
7 Liquid Chromatography‐Mass Spectrometry (LC‐MS) Quantification of Reactive Metabolites
7.1 Introduction
7.2 LC‐MS Methods Using GSH as a Trapping Reagent
7.2.1 LC‐MS Approaches at Positive Mode Using Constant Neutral Loss (CNL) Scan or Enhanced Product Ion (EPI) Scan
7.2.2 LC‐MS Approaches at Negative Mode Using Neutral Loss, Pre‐Ion Scan (PIS) and XoPI (Extraction of Product Ion)
7.2.3 LC‐MS Approaches Using Stable Isotopic‐GSH
7.2.4 LC‐MS Approaches Using Combined XoPI and Stable‐Isotopic GSH
7.2.5 LC‐MS Coupled with Software‐Assisted Approach
7.2.6 Using GSH Derivatives as Trapping Reagents for Detection and Quantitation
7.3 Using Other Trapping Reagents
7.4 Identification and Characterization of Rearranged GSH Adducts
7.5 Strategies for Optimization and Decision Tree
7.6 Summary
Acknowledgment
Abbreviations
References
8 Human‐Based In Vitro Experimental Approaches for the Evaluation of Metabolism‐Dependent Drug Toxicity
8.1 Introduction
8.2 Assays for Reactive Metabolites
8.2.1 Glutathione Trapping Assay
8.2.2 Covalent Binding Assay
8.3 Cell‐Based Assays for Metabolism‐Dependent Toxicity
8.4 Primary Human Hepatocyte Assays for Metabolism‐Dependent Drug Toxicity
8.4.1 In Vitro Screening Assays for Hepatotoxicity
8.4.2 Cytotoxic Metabolic Pathway Identification Assay (CMPIA)
8.4.3 Metabolic Comparative Cytotoxicity Assay (MCCA)
8.4.4 MetMax™ Cryopreserved Human Hepatocytes (MMHH) Metabolic Activation Cytotoxicity Assay (MMACA)
8.5 Emerging Hepatocyte Technologies for the Evaluation of Drug Toxicity. 8.5.1 Human Hepatocytes ROS/ATP Assay for DILI Drugs
8.5.2 Long‐Term Hepatocyte Cultures. 8.5.2.1 999Elite™ Long‐Term Cultured Human Hepatocytes
8.5.2.2 Hepatocyte/Non‐Hepatocyte Cocultures
8.5.2.3 Human Hepatocyte Spheroids
8.5.2.4 Microfluidic 3‐Dimensional (3‐d) Hepatocyte Cultures
8.6 Integrated Discrete Multiple Organ Coculture (IdMOC®)
8.7 Conclusion
References
9 Mechanism‐Based Experimental Models for the Evaluation of BSEP Inhibition and DILI
9.1 Introduction. 9.1.1 Drug‐Induced Liver Injury
9.1.2 Bile Acid Homeostasis and Role of Bile Salt Export Pump
9.2 Membrane Vesicles to Study BSEP Inhibition
9.2.1 Membrane Vesicle Preparations
9.2.2 Membrane Vesicle Assays and Data Interpretation
9.3 Sandwich‐Cultured Hepatocytes to Study BSEP Inhibition. 9.3.1 B‐CLEAR® Assay
9.3.2 Uptake and Efflux Studies with Mechanistic Modeling
9.4 Other In Vitro Methods to Study BSEP Inhibition
9.5 Computational Methods Used to Predict BSEP Inhibition
9.6 In Vitro Models as a Predictor of Clinical DILI. 9.6.1 The C‐DILI™ Assay
9.7 Preclinical In Vivo Models for the Evaluation of BSEP Inhibition and DILI
9.8 In Vivo Clinical Biomarkers of BSEP Inhibition and DILI
9.8.1 Serum Bile Acids as Clinical Biomarkers
9.8.2 Clinical Biomarkers of DILI
9.9 Quantitative Systems Toxicology to Predict DILI
9.10 Conclusions
Funding Information
Conflict of Interest
Acknowledgments
References
10 Hepatic Bile Acid Transporters in Drug‐Induced Cholestasis
Abbreviations
10.1 Introduction
10.2 Bile Acid and DIC
10.2.1 Bile Acid
10.2.1.1 Bile Acid Synthesis
10.2.1.2 Bile Acid Transport
10.2.2 Cytotoxicity of Bile Acids and DIC
10.3 Hepatic Bile Acid Uptake Transporters in DIC
10.3.1 Sodium‐Taurocholate Cotransporting Polypeptide (NTCP)
10.3.1.1 Substrates of NTCP
10.3.1.2 Regulation of NTCP
10.3.1.3 NTCP and Cholestasis
10.3.2 Other Hepatic Bile Acid Uptake Transporters
10.4 Hepatic Bile Acid Efflux Transporters in DIC
10.4.1 Bile Salt Export Pump (BSEP)
10.4.1.1 Substrates of BSEP
10.4.1.2 Regulation of BSEP
10.4.1.3 Internalization of BSEP
10.4.1.4 BSEP and Cholestasis
10.4.2 Other Hepatic Bile Acid Efflux Transporters
10.4.2.1 MRP2
10.4.2.2 MRP3 and MRP4
10.5 Bidirectional Bile Acid Transporter OSTα/β
10.6 Summary
References
11 Role of Renal Transporters in Drug–Drug Interactions and Nephrotoxicity
11.1 Overview of Renal Transporters
11.1.1 Basolateral Transporters
11.1.2 Apical Transporters
11.2 Renal Transporters and Drug–Drug Interactions
11.2.1 Impact on the Pharmacokinetics of Drugs
11.2.2 Impact on the Drug PD
11.3 Renal Transporters and Nephrotoxicity
11.3.1 Nephrotoxicity Unrelated to Drug Transporters
11.3.2 Nephrotoxicity Related to Drug Transporters
11.4 Biomarkers and Nephrotoxicity. 11.4.1 Biomarkers for Detecting Glomerular Injury
11.4.2 Biomarkers for Drug‐Induced Injury to Proximal and Distal Tubules
11.5 Conclusion
References
12 Blood–Brain Barrier Transporters and Central Nervous System Drug Response and Toxicity
12.1 Over‐View of the Brain Barriers
12.1.1 Blood–Brain Barrier (BBB)
12.1.2 Blood–Cerebrospinal Fluid Barrier (BCSFB)
12.1.3 CSF as Predictor of Drug Exposure in the Brain
12.1.4 Solute Carriers in the BBB
12.1.5 Drug Efflux Transporters in the BBB
12.2 General Influence of BBB Transporters on Drug Entry into the Brain
12.3 BBB‐Transporter Effects on CNS Drug Response
12.3.1 Influence of Efflux Transporters on Brain Disposition of Drugs. 12.3.1.1 Anticancer Agents
12.3.1.2 Opioids
12.3.2 SLCs and BBB Transport of Drugs
12.4 Transporter Considerations Influencing CNS Drug Response
12.4.1 Transporter Polymorphisms. 12.4.1.1 P‐gp Polymorphism
12.4.1.2 BCRP Polymorphism
12.4.1.3 SLC Polymorphism
12.4.2 Age‐Related Alterations in BBB Transporter Function and Drug Response
12.4.3 Disease‐Dependent Modulation of BBB Transporters and Drug Response. 12.4.3.1 Inflammation and Pain
12.4.3.2 Epilepsy
12.4.4 CNS Toxicity Caused by Drug Interactions at the BBB
12.5 Conclusions
References
13 Ototoxicity and Drug Transport in the Cochlea
13.1 Auditory System Anatomy
13.1.1 External, Middle, and Inner Ear
13.1.1.1 Anatomy of the Inner Ear
13.1.1.2 Hair Cell Anatomy
13.1.2 Blood–Labyrinth Barrier
13.2 Auditory System Physiology
13.3 Hearing Loss, Ototoxic Drugs, and Hair Cell Damage
13.3.1 Aminoglycosides
13.3.2 Platinum Chemotherapeutics
13.3.3 Salicylate
13.4 Drug Metabolism in the Ear. 13.4.1 The Importance of Drug Metabolism in the Ear
13.4.2 Studies of Drug‐Metabolizing Enzymes in Ototoxicity
13.4.3 Drug Transporters in the Ear
13.5 Conclusion
References
14 Application of a PBPK Model Incorporating the Interplay Between Transporters and Drug‐Metabolizing Enzymes for the Precise Prediction of Drug Toxicity
14.1 Importance of the Consideration of Intracellular Concentration of Drugs in the Tissue for Estimation of Pharmacological/Toxicological Effects of Drugs
14.2 Extended Clearance Concept as a Tool to Explain Theoretically Transporter and Drug‐Metabolizing Enzyme Interplay
14.3 Theoretical Consideration of the Intracellular Concentration of Drugs in the Tissue
14.4 The Benefits of Using a PBPK Model for the Accurate Prediction of Pharmacological/Toxicological Effects of Drugs
14.5 VCT to Simulate the Distribution of Clinical Outcomes in a Specific Population with Defined Mean and Variability of Parameters in a PBPK Model
14.5.1 VCT of Docetaxel to Estimate the Effects on the Risk of Neutropenia of Genetic Polymorphisms in OATP1B3 and MRP2
14.5.2 VCT of Oseltamivir and Its Active Metabolite (Ro 64‐0802) to Estimate the Effects on Their Brain Exposure of Genetic Polymorphisms in Multiple Uptake/Efflux Transporters
14.5.3 VCT of Irinotecan and Its Metabolites to Estimate the Effects of Genetic Polymorphisms in Multiple Uptake/Efflux Transporters on Irinotecan‐Induced Side Effects (Neutropenia, Diarrhea)
14.6 Conclusions and Future Perspectives
References
15 The Extended Clearance Model : A Valuable Tool For Drug‐Induced Liver Injury Risk Prediction
15.1 Introduction
15.2 Application of the ECM to Estimate Kpuu Liver
15.2.1 Introduction to the ECM: Concepts and Application for the Prediction of Hepatic Clearance and Drug–Drug Interactions
15.2.2 Concept of Kpuu Liver
15.2.3 Estimation of Kpuu Liver from In Vitro Data Using the ECM
15.3 Relevant Concentrations for the DILI Risk Assessment
15.3.1 Maximum Plasma Concentrations
15.3.2 Maximum Hepatic Inlet Concentrations
15.3.3 Maximum Intracellular Hepatocyte Concentrations
15.4 Assessing the DIC Risk Using ECM‐Based Unbound Intrahepatic Concentrations and Accounting for BSEP Inhibition as a Single Mechanism
15.5 Assessing the DILI Risk Using the “1/R‐Value Model” to Account for the Inhibition of Multiple Pathways
15.5.1 ECM‐Based 1/R‐Value Model
15.5.2 1/R vs Safety Margin Relationship
15.6 Discussion and Outlook
References
Index
a
b
c
d
e
f
g
h
i
k
l
m
n
o
p
q
r
s
t
u
v
w
x
WILEY END USER LICENSE AGREEMENT
Отрывок из книги
Edited by Albert P. Li
.....
Following in vitro incubations, various analytical approaches such as LC‐MS, fluorescence or radiochemical detection could be used to analyze the trapped adducts and/or conjugates of RMs. Mass spectrometric methods are the most common approach. Taking advantage of specific fragmentation behavior of the peptide moiety of nucleophilic trapping reagents, stable trapping adducts, and/or conjugates of electrophilic RMs can be detected and characterized. For example, the neutral loss of 129 Da is commonly used by positive ion electrospray‐tandem mass spectrometry to provide a generic endpoint for GSH trapping and measurement. Without using the radiolabeled drugs, this protocol can provide semi‐quantitative estimates of adduct formation for RMs.
Occasionally the formed RMs (electrophiles) are so highly reactive that they cannot flow out from the active site of the P450 enzymes that catalyzed their formation. Electrophilic intermediates derived from drug molecules are so reactive that they can covalently bind directly to an active site amino acid residue in the CYP enzyme itself. Covalent modification in the active site of a P450 enzyme may lead to the loss of enzyme activity over time via the modification of the P450 apoprotein. Therefore, time dependent inactivation (TDI) of CYP450 enzymes and other drug‐metabolizing enzymes also indicates the generation of RMs during the drug metabolism process. Additionally, drug–drug interactions could be caused by this irreversible P450 inhibition. Covalent modification of P450 enzymes can also result in a neoantigen formation and trigger an autoimmune response in DILI.
.....