Solar-to-Chemical Conversion
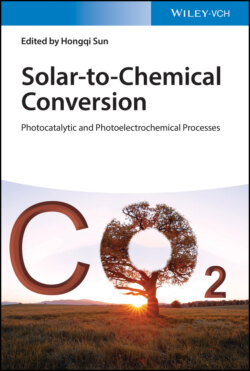
Реклама. ООО «ЛитРес», ИНН: 7719571260.
Оглавление
Группа авторов. Solar-to-Chemical Conversion
Contents
List of Tables
List of Illustrations
Guide
Pages
Solar‐to‐Chemical Conversion. Photocatalytic and Petrochemical Processes
1. Introduction: A Delicate Collection of Advances in Solar‐to‐Chemical Conversions
2. Artificial Photosynthesis and Solar Fuels
2.1 Introduction of Solar Fuels
2.2 Photosynthesis. 2.2.1 Natural Photosynthesis
2.2.2 Artificial Photosynthesis
2.3 Principles of Photocatalysis
2.4 Products of Artificial Photosynthesis. 2.4.1 Hydrocarbons
2.4.1.1 Methane (CH4)
2.4.1.2 Methanol (CH3OH)
2.4.1.3 Formaldehyde (HCHO)
2.4.1.4 Formic Acid (HCOOH)
2.4.1.5 C2 Hydrocarbons
2.4.1.6 Other Hydrocarbons
2.4.2 Carbon Monoxide (CO)
2.4.3 Dioxygen (O2)
2.5 Perspective
Acknowledgments
References
3. Natural and Artificial Photosynthesis
3.1 Introduction
3.2 Overview of Natural Photosynthesis
3.3 Light Harvesting and Excitation Energy Transfer
3.4 Charge Separation and Electron Transfer
3.5 Water Oxidation
3.6 Carbon Fixation
3.7 Conclusions
References
4. Photocatalytic Hydrogen Evolution
4.1 Introduction
4.2 Fundamentals of Photocatalytic H2 Evolution
4.3 Photocatalytic H2 Evolution Under UV Light. 4.3.1 Titanium Dioxide (TiO2)‐Based Semiconductors
4.3.2 Other Types of UV‐Responsive Photocatalysts
4.4 Photocatalytic H2 Evolution Under Visible Light. 4.4.1 Carbon Nitride (C3N4)‐Based Semiconductor
4.4.2 Other Types of Visible‐Light‐Responsive Photocatalysts
4.5 Photocatalytic H2 Evolution Under Near‐Infrared Light
4.6 Roles of Sacrificial Reagents and Reaction Pathways
4.7 Summary and Outlook
References
5. Photoelectrochemical Hydrogen Evolution
5.1 Background of Photoelectrocatalytic Water Splitting
5.2 Mechanism of Charge Separation and Transfer
5.3 Strategy for Improving Charge Transfer
5.3.1 Improving the Charge Transfer in Continuous Film
5.3.2 Improving the Charge Transfer in Particulate Photoelectrodes
5.4 Strategy for Improving Electron–Hole Separation
5.4.1 Heterojunction Formation
5.4.2 Crystal Facet Control
5.4.3 Surface Passivation
5.5 Surface Cocatalyst Design
5.6 Unbiased PEC Water Splitting
5.7 Conclusion and Perspective
References
6. Photocatalytic Oxygen Evolution
6.1 Introduction. 6.1.1 Configuration of Photocatalytic Water Oxidation
6.1.2 Mechanism, Thermodynamics, and Kinetics Toward Efficient Oxygen Evolution
6.2 Homogeneous Photocatalytic Water Oxidation. 6.2.1 Molecular Complexes and Polyoxometalates
6.2.2 Mechanism Details and the Stability
6.3 Heterogeneous Photocatalytic Water Oxidation
6.3.1 Unique Properties of Nanosized Semiconductor System. 6.3.1.1 Quantum Confinement
6.3.1.2 Localized Surface Plasmon Resonance (LSPR)
6.3.1.3 Surface Area and Exposed Facet‐Enhanced Charge Transfer
6.3.2 Zero‐Dimensional Semiconductor Materials for Photocatalytic Water Oxidation
6.3.2.1 0D Metal Complexes and Nanoclusters
6.3.2.2 Metal Oxide Quantum Dots and Nanocrystals
6.3.3 One‐Dimensional Semiconductor Materials for Photocatalytic Water Oxidation
6.3.4 Two‐Dimensional Semiconductor Materials for Photocatalytic Water Oxidation
6.3.4.1 2D Metal Oxide Nanosheets for Photocatalytic Water Oxidation
6.3.4.2 Layered Double Hydroxide (LDH) Nanosheets for Photocatalytic Water Oxidation
6.3.4.3 Metal‐Based Oxyhalide Semiconductors for Photocatalytic Water Oxidation
6.3.5 LD Semiconductor‐Based Hybrids for Photocatalytic Oxygen Evolution
6.3.5.1 1D‐Based (0D/1D and 1D/1D) Semiconductor Hybrids for Enhanced Photocatalytic Water Oxidation
6.3.5.2 2D‐Based (2D/2D) Semiconductor Hybrids for Enhanced Photocatalytic Water Oxidation
6.3.5.3 Metal‐Free‐Based Semiconductors for Water Oxidation
6.4 Catalytic Active Site–Catalysis Correlation in LD Semiconductors
6.5 Conclusions and Perspectives
References
7 Photoelectrochemical Oxygen Evolution
7.1 Introduction
7.2 Honda–Fujishima Effect
7.3 Factors Affecting the Photoanodic Current
7.4 Electrode Potentials at Different pH
7.5 Evaluation of PEC Performance
7.6 Flat Band Potential and Photocurrent Onset Potential
7.7 Selection of Materials
7.8 Enhancement of PEC Properties
7.8.1 Nanostructuring and Morphology Control
7.8.2 Donor Doping
7.8.3 Modification of Photoanode Surface
7.8.4 Electron‐Conductive Materials
7.9 PEC Device for Water Splitting
7.10 Conclusions and Outlook
References
8. Photocatalytic and Photoelectrochemical Overall Water Splitting
8.1 Introduction
8.2 Photocatalytic Overall Water Splitting
8.2.1 Principles and Mechanism
8.2.2 Key Performance Indicators
8.2.3 Materials for One‐Step Photoexcitation Toward Overall Water Splitting
8.2.3.1 Semiconductors. Oxides
Nitrides
Oxynitrides
Metal Chalcogenides
Carbon‐Based Materials
8.2.3.2 Incorporation of Cocatalysts
8.2.3.3 Plasmonic Nanostructures
8.2.4 Hybrid Systems for Two‐Step Photoexcitation Toward Overall Water Splitting
8.2.4.1 Z‐Schemes
First‐Generation Z‐Schemes (Liquid Phase)
Second‐Generation Z‐Schemes (All Solid State)
Third‐Generation Z‐Scheme (Direct Z‐Scheme)
8.3 Photoelectrochemical Overall Water Splitting
8.3.1 Principles and Mechanism
8.3.2 Key Performance Indicators
8.3.3 Materials Design
8.3.3.1 Photoanode Materials
8.3.3.2 Photocathode Materials
8.3.4 Unassisted Photoelectrochemical Overall Water Splitting
8.3.4.1 Photoanode–Photocathode Tandem Cells
8.3.4.2 Photovoltaic–Photoelectrode Devices
Non‐artificial PV–PEC Devices
Artificial (Wireless) PV–PEC Devices
8.4 Concluding Remarks and Outlook
Acknowledgments
References
9. Photocatalytic CO2 Reduction
9.1 Introduction
9.2 Principle of Photocatalytic Reduction of CO2
9.3 Energy and Mass Transfers in Photocatalytic Reduction of CO2
9.3.1 Energy Flow from the Concentrator to Reactor
9.3.2 Energy Flow on the Surface of the Photocatalyst
9.3.3 Mass Flow in CO2 Photocatalytic Reduction
9.3.4 Product Selectivity in CO2 Photocatalytic Reaction
9.4 Conclusions
Acknowledgments
References
10. Photoelectrochemical CO2 Reduction
10.1 Introduction
10.1.1 Introduction of Photoelectrocatalytic Reduction of CO2
10.1.2 Principles of Photoelectrocatalytic Reduction of CO2
10.1.3 System Configurations of Photoelectrocatalytic Reduction of CO2
10.2 PEC CO2 Reduction Principles. 10.2.1 Thermodynamics and Kinetics of CO2 Reduction
10.2.2 Reaction Conditions
10.2.2.1 Reaction Temperature and Pressure
10.2.2.2 pH Value
10.2.2.3 Solvent
10.2.2.4 External Electrical Bias
10.2.3 Performance Evaluation of PEC CO2 Reduction
10.2.3.1 Product Evolution Rate and Catalytic Current Density
10.2.3.2 Turnover Number and Turnover Frequency
10.2.3.3 Overpotential
10.2.3.4 Faradaic Efficiency
10.3 Application of Solar‐to‐Chemical Energy Conversion in PEC CO2 Reduction
10.3.1 PEC CO2 Reduction on Semiconductors
10.3.1.1 Oxide Semiconductors
10.3.1.2 Non‐oxide Semiconductors
10.3.1.3 Chalcogenide Semiconductors
10.3.2 PEC CO2 Reduction on Cocatalyst Systems
10.3.2.1 Metal Nanoparticles
10.3.2.2 Metal Complexes
10.3.3 PEC CO2 Reduction on Hybrid Semiconductors
10.3.3.1 Conductive Polymers
10.3.3.2 Enzymatic Biocatalysts
10.3.3.3 Organic Molecules
10.4 Other Configurations for PEC CO2 Reduction
10.5 Conclusion and Outlook
Acknowledgments
Conflict of Interest
References
11. Photocatalytic and Photoelectrochemical Nitrogen Fixation
11.1 Introduction
11.2 Fundamental Principles and Present Challenges. 11.2.1 Principles in N2 Reduction for NH3 Production
11.2.2 Challenges for N2 Reduction to NH3
11.3 Strategies for Catalyst Design and Fabrication. 11.3.1 Defect Engineering
11.3.1.1 Vacancies
Oxygen Vacancies
Nitrogen Vacancies
Sulfur Vacancies
11.3.1.2 Heteroatom Doping
11.3.1.3 Amorphization
11.3.2 Structure Engineering. 11.3.2.1 Morphology Regulation
11.3.2.2 Facet Control
11.3.3 Interface Engineering
11.3.4 Heterojunction Engineering
11.3.5 Co‐catalyst Engineering
11.3.6 Biomimetic Engineering
11.4 Conclusions and Outlook
References
12. Photocatalytic Production of Hydrogen Peroxide Using MOF Materials
12.1 Introduction
12.2 Photocatalytic H2O2 Production Through Selective Two‐Electron Reduction of O2 Utilizing NiO/MIL‐125‐NH2
12.3 Two‐Phase System Utilizing Linker‐Alkylated Hydrophobic MIL‐125‐NH2 for Photocatalytic H2O2 Production
12.4 Ti Cluster‐Alkylated Hydrophobic MIL‐125‐NH2 for Photocatalytic H2O2 Production in Two‐Phase System
12.5 Conclusion and Outlooks
Reference
13. Photocatalytic and Photoelectrochemical Reforming of Methane
13.1 Introduction
13.2 Photo‐Mediated Processes
13.3 Differences Between Photo‐Assisted Catalysis and Thermocatalysis
13.3.1 Catalyst Involved
13.3.2 Reactors
13.3.3 Mechanism
13.3.4 Equations for Quantum Efficiency
13.4 Reactions of Methane Conversion via Photo‐Assisted Catalysis
13.4.1 Methane Dry Reforming
13.4.2 Methane Steam Reforming
13.4.3 Methane Coupling
13.4.4 Methane Oxidation
13.4.5 Methane Dehydroaromatization
13.5 Conclusions and Perspectives
Acknowledgment
References
14. Photocatalytic and Photoelectrochemical Reforming of Biomass
14.1 Introduction
14.2 Fundamentals of Photocatalytic and Photoelectrochemical Processes. 14.2.1 Photocatalytic Process
14.2.2 Photoelectrochemical Process
14.3 Photocatalytic Reforming of Biomass. 14.3.1 Photocatalytic Reforming of Lignin
14.3.2 Photocatalytic Reforming of Carbohydrates
14.3.3 Photocatalytic Reforming of Native Lignocellulose
14.3.4 Photocatalytic Reforming of Triglycerides and Glycerol
14.4 Photoelectrochemical Reforming of Biomass
14.4.1 Photoelectrochemical Conversion of Biomass to Produce Electricity
14.4.2 Photoelectrochemical Conversion of Biomass to Produce Hydrogen
14.4.3 Photoelectrochemical Conversion of Biomass to Produce Chemicals
14.5 Conclusion Remarks and Perspectives
Acknowledgments
References
15 Reactors, Fundamentals, and Engineering Aspects for Photocatalytic and Photoelectrochemical Systems
15.1 Fundamental Mechanisms of Photocatalytic and PEC Processes
15.1.1 Rationales of Photocatalytic Systems
15.1.1.1 Photocatalytic Water Splitting
15.1.1.2 Photocatalytic CO2 reduction
15.1.2 Rationales of PEC Systems
15.2 Reactor Design and Configuration
15.2.1 Reactors for Photocatalytic Systems. 15.2.1.1 Reactors for Photocatalytic Water Splitting
15.2.1.2 Reactors for Photocatalytic CO2 Reduction
15.2.2 Reactors for PEC Systems
15.3 Engineering Aspects of Photocatalytic and PEC Processes
15.3.1 Photocatalyst Sheets: Scaling‐up of Photocatalytic Water Splitting
15.3.2 Monolithic Devices: Wireless Approach of PEC Reaction
15.4 Conclusions and Outlook
Acknowledgments
List of Abbreviations
References
16 Prospects of Solar Fuels
Index. a
b
c
d
e
f
g
h
i
k
l
m
n
o
p
q
r
s
t
u
v
w
x
z
WILEY END USER LICENSE AGREEMENT
Отрывок из книги
Edited by Hongqi Sun
Hongqi Sun
.....
Figure 3.6 Simplified Z‐scheme of natural oxygenic photosynthesis, showing how two photons are used per electron flowing from the terminal donor (H2O) to the terminal acceptor (NADP+) of the light‐dependent reactions.
Both photosystems have homodimeric structures and exhibit high similarity in the proteins and cofactors comprising their core regions, suggestive of their common evolutionary origin. In the following we will focus on the enzyme responsible for water oxidation, PS‐II (see Figure 3.7). Crystallographic structures of PS‐II are mostly available from thermophilic cyanobacteria such as Thermosynechococcus elongatus and Thermosynechococcus vulcanus. Conventional X‐ray diffraction (XRD) studies, which first yielded a PS‐II crystallographic model in 2001 [47] and make use of synchrotron X‐ray radiation, have more recently been supplanted by approaches that utilize X‐ray free‐electron laser (XFEL) femtosecond pulses [48, 49]. Through a long series of XRD studies [50–56], the highest‐resolution cyanobacterial PS‐II crystallographic models currently stand at 1.9 Å [55] and 1.87/1.85 Å [56]. Presently available XFEL models have still not achieved comparable resolution, but they have opened the way for probing intermediate states of the water oxidation cycle [57-63]. Higher‐plant PS‐II structures that resolve internal cofactors have so far been reported from cryo‐electron microscopy at comparatively lower resolution [64, 65].
.....