Fundamentals of Terahertz Devices and Applications
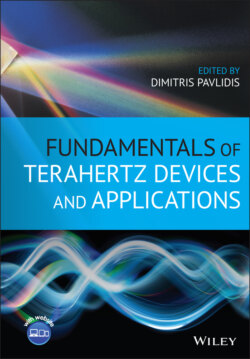
Реклама. ООО «ЛитРес», ИНН: 7719571260.
Оглавление
Группа авторов. Fundamentals of Terahertz Devices and Applications
Table of Contents
List of Tables
List of Illustrations
Guide
Pages
Fundamentals of Terahertz Devices and Applications
About the Editor
List of Contributors
About the Companion Website
1 Introduction to THz Technologies
2 Integrated Silicon Lens Antennas at Submillimeter‐wave Frequencies
2.1 Introduction
2.2 Elliptical Lens Antennas
2.2.1 Elliptical Lens Synthesis
2.2.2 Radiation of Elliptical Lenses
2.2.2.1 Transmission Function
2.2.2.2 Spreading Factor S(Q)
2.2.2.3 Equivalent Current Distribution and Far‐field Calculation
2.2.2.4 Lens Reflection Efficiency
2.3 Extended Semi‐hemispherical Lens Antennas
2.3.1 Radiation of Extended Semi‐hemispherical Lenses
2.4 Shallow Lenses Excited by Leaky Wave/Fabry–Perot Feeds
2.4.1 Analysis of the Leaky‐wave Propagation Constant
2.4.2 Primary Fields Radiated by a Leaky‐wave Antenna Feed on an Infinite Medium
2.4.3 Shallow‐lens Geometry Optimization
2.5 Fly‐eye Antenna Array
2.5.1 Silicon DRIE Micromachining Process at Submillimeter‐wave Frequencies
2.5.1.1 Fabrication of Silicon Lenses Using DRIE
2.5.1.2 Surface Accuracy
2.5.2 Examples of Fabricated Antennas
Exercises. E2.1 Derivation of the Transmission Coefficients and Lens Critical Angle
E2.2
E2.3
References
3 Photoconductive THz Sources Driven at 1550 nm
3.1 Introduction. 3.1.1 Overview of THz Photoconductive Sources
3.1.2 Lasers and Fiber Optics
3.2 1550‐nm THz Photoconductive Sources. 3.2.1 Epitaxial Materials. 3.2.1.1 Bandgap Engineering
3.2.1.2 Low‐Temperature Growth
3.2.2 Device Types and Modes of Operation
3.2.3 Analysis of THz Photoconductive Sources
3.2.3.1 PC‐Switch Analysis
3.2.3.2 Photomixer Analysis
3.2.3.2.1 p–i–n Photodiode
3.2.3.2.2 MSM Bulk Photoconductor
3.2.4 Practical Issues
3.2.4.1 Contact Effects
3.2.4.2 Thermal Effects
3.2.4.3 Circuit Limitations
3.3 THz Metrology. 3.3.1 Power Measurements. 3.3.1.1 A Traceable Power Sensor
3.3.1.2 Exemplary THz Power Measurement Exercise
3.3.1.3 Other Sources of Error
3.3.2 Frequency Metrology
3.4 THz Antenna Coupling. 3.4.1 Fundamental Principles
3.4.2 Planar Antennas on Dielectric Substrates
3.4.2.1 Input Impedance
3.4.2.2 ΔEIRP (Increase in the EIRP of the Transmitting Antenna)
3.4.2.3 G/T or Aeff/T
3.4.3 Estimation of Power Coupling Factor
3.4.4 Exemplary THz Planar Antennas
3.4.4.1 Resonant Antennas
3.4.4.2 Quick Survey of Self‐complementary Antennas
3.4.4.2.1 Bow‐Tie Antennas
3.4.4.2.2 Log‐Spiral Antennas
3.4.4.2.3 Log‐Periodic Antennas
3.5 State of the Art in 1550‐nm Photoconductive Sources. 3.5.1 1550‐nm MSM Photoconductive Switches. 3.5.1.1 Material and Device Design
3.5.1.2 THz Performance
3.5.2 1550‐nm Photodiode CW (Photomixer) Sources. 3.5.2.1 Material and Device Design
3.5.2.2 THz Performance
3.6 Alternative 1550‐nm THz Photoconductive Sources
3.6.1 Fe‐Doped InGaAs
3.6.2 ErAs Nanoparticles in GaAs: Extrinsic Photoconductivity
3.7 System Applications. 3.7.1 Comparison Between Pulsed and CW THz Systems. 3.7.1.1 Device Aspects
3.7.1.2 Systems Aspects
3.7.2 Wireless Communications
3.7.3 THz Spectroscopy. 3.7.3.1 Time vs Frequency Domain Systems
3.7.3.2 Analysis of Frequency Domain Systems: Amplitude and Phase Modulation
Exercises
Exercise: THz Interaction with Matter
Exercise: Antennas, Links, and Beams
Exercise: Planar Antennas
Exercise: Device Noise, System Noise, and Dynamic Range
Exercise: Ultrafast Photoconductivity and Photodiodes
References
Explanatory Notes (see superscripts in text)
4 THz Photomixers
4.1 Introduction
4.2 Photomixing Basics. 4.2.1 Photomixing Principle
4.2.2 Historical Background
4.3 Modeling THz Photomixers
4.3.1 Photoconductors. 4.3.1.1 Photocurrent Generation
4.3.1.2 Electrical Model
4.3.1.3 Efficiency and Maximum Power. 4.3.1.3.1 Case G1 = G0 and C = 0
4.3.1.3.2 General Case
4.3.2 Photodiode. 4.3.2.1 PIN photodiodes
4.3.2.2 Uni‐Traveling‐Carrier Photodiodes
4.3.2.3 Photocurrent Generation
4.3.2.4 Electrical Model and Output Power
4.3.3 Frequency Down‐conversion Using Photomixers
4.3.3.1 Electrical Model: Conversion Loss
4.4 Standard Photomixing Devices. 4.4.1 Planar Photoconductors
4.4.1.1 Intrinsic Limitation
4.4.2 UTC Photodiodes. 4.4.2.1 Backside Illuminated UTC Photodiodes
4.4.2.2 Waveguide‐fed UTC Photodiodes
4.5 Optical Cavity Based Photomixers. 4.5.1 LT‐GaAs Photoconductors
4.5.1.1 Optical Modeling
4.5.1.2 Experimental Validation. 4.5.1.2.1 Photoresponse
4.5.1.2.2 Photomixing Experiment in the 220–325 GHz Frequency Band. 4.5.1.2.2.1 Photomixer Characteristics
4.5.1.2.2.2 Experimental Set‐up
4.5.1.2.2.3 Results
4.5.1.2.3 Output Power Estimation at 1 THz
4.5.1.2.4 Frequency Down Conversion Using An Optical Cavity LT‐GaAs Photoconductor
4.5.2 UTC Photodiodes
4.5.2.1 Nano Grid Top Contact Electrodes
4.5.2.2 UTC Photodiodes Using Nano‐Grid Top Contact Electrodes
4.5.2.3 Photoresponse Measurement
4.5.2.4 THz Power Generation by Photomixing
4.6 THz Antennas
4.6.1 Planar Antennas
4.6.2 Micromachined Antennas
4.7 Characterization of Photomixing Devices. 4.7.1 On Wafer Characterization
4.7.2 Free Space Characterization
Exercises. Exercise A. Photodetector Theory
Exercise B. Photomixing Model. 1. Ultrafast Photoconductor
2. UTC Photodiode
Exercise C. Antennas
References
5 Plasmonics‐enhanced Photoconductive Terahertz Devices
5.1 Introduction
5.2 Photoconductive Antennas. 5.2.1 Photoconductors for THz Operation
5.2.2 Photoconductive THz Emitters
5.2.2.1 Pulsed THz Emitters
5.2.2.2 Continuous‐wave THz Emitters
5.2.3 Photoconductive THz Detectors
5.2.4 Common Photoconductors and Antennas for Photoconductive THz Devices. 5.2.4.1 Choice of Photoconductor
5.2.4.2 Choice of Antenna
5.3 Plasmonics‐enhanced Photoconductive Antennas. 5.3.1 Fundamentals of Plasmonics
5.3.2 Plasmonics for Enhancing Performance of Photoconductive THz Devices. 5.3.2.1 Principles of Plasmonic Enhancement
5.3.2.2 Design Considerations for Plasmonic Nanostructures
5.3.3 State‐of‐the‐art Plasmonics‐enhanced Photoconductive THz Devices. 5.3.3.1 Photoconductive THz Devices with Plasmonic Light Concentrators
5.3.3.2 Photoconductive THz Devices with Plasmonic Contact Electrodes
5.3.3.3 Large Area Plasmonic Photoconductive Nanoantenna Arrays
5.3.3.4 Plasmonic Photoconductive THz Devices with Optical Nanocavities
5.4 Conclusion and Outlook
Exercises
References
6 Terahertz Quantum Cascade Lasers
6.1 Introduction
6.2 Fundamentals of Intersubband Transitions
6.3 Active Material Design
6.4 Optical Waveguides and Cavities
6.5 State‐of‐the‐Art Performance and Limitations
6.6 Novel Materials Systems
6.6.1 III‐Nitride Quantum Wells
6.6.2 SiGe Quantum Wells
6.7 Conclusion
Acknowledgments
Exercises
References
7 Advanced Devices Using Two‐Dimensional Layer Technology
7.1 Graphene‐Based THz Devices
7.1.1 THz Properties of Graphene
7.1.2 How to Simulate and Model Graphene?
7.1.3 Terahertz Device Applications of Graphene
7.1.3.1 Modulators. 7.1.3.1.1 Broadband Structures
7.1.3.1.2 Electromagnetic‐cavity Integrated Structures
7.1.3.1.3 Graphene/Metal‐Hybrid Metamaterials
7.1.3.1.4 Graphene/Dielectric‐Hybrid Metamaterials
7.1.3.2 Active Filters
7.1.3.3 Phase Modulation in Graphene‐Based Metamaterials
7.2 TMD Based THz Devices
7.3 Applications
Exercises
E7.1 Computation of the Optical Conductivity of Graphene
E7.2 Terahertz Transmission Through a 2D Material Layer Placed at an Optical Interface
E7.3 Transfer Matrix Approach for Multi‐layer Transmission Problems
E7.4 A Condition for Perfect Absorption
E7.5 Terahertz Plasmon Resonances in Periodically Patterned Graphene Disk Arrays
E7.6 Electron Plasma Waves in Gated Graphene
E7.7 Equivalent Circuit Modeling of 2D Material‐Loaded Frequency Selective Surfaces
E7.8 Maximum Terahertz Absorption in 2D Material‐Loaded Frequency Selective Surfaces
References
8 THz Plasma Field Effect Transistor Detectors
8.1 Introduction
8.2 Field Effect Transistors (FETs) and THz Plasma Oscillations
8.2.1 Dispersion of Plasma Waves in FETs
8.2.2 THz Detection by an FET
8.2.2.1 Resonant Detection
8.2.2.2 Broadband Detection
8.2.2.3 Enhancement by DC Drain Current
8.3 THz Detectors Based on Silicon FETs
8.4 Terahertz Detection by Graphene Plasmonic FETs
8.5 Terahertz Detection in Black‐Phosphorus Nano‐Transistors
8.6 Diamond Plasmonic THz Detectors
8.7 Conclusion
Exercises
References
9 Signal Generation by Diode Frequency Multiplication
9.1 Introduction
9.2 Bridging the Microwave to Photonics Gap with Terahertz Frequency Multipliers
9.3 A Practical Approach to the Design of Frequency Multipliers. 9.3.1 Frequency Multiplier Versus Comb Generator
9.3.2 Frequency Multiplier Ideal Matching Network and Ideal Device Performance
9.3.3 Symmetry at Device Level Versus Symmetry at Circuit Level
9.3.4 Classic Balanced Frequency Doublers
9.3.4.1 General Circuit Description
9.3.4.2 Necessary Condition to Balance the Circuit
9.3.5 Balanced Frequency Triplers with an Anti‐Parallel Pair of Diodes
9.3.6 Multi‐Anode Frequency Triplers in a Virtual Loop Configuration
9.3.6.1 General Circuit Description
9.3.6.2 Necessary Condition to Balance the Circuit
9.3.7 Multiplier Design Optimization
9.3.7.1 General Design Methodology
9.3.7.1.1 Bias Voltage
9.3.7.1.2 Input Power
9.3.7.1.3 Epitaxial Layer Length and Doping
9.3.7.1.4 Anode Area
9.3.7.1.5 Temperature
9.3.7.1.6 Carrier Velocity Saturation
9.3.7.2 Nonlinear Modeling of the Schottky Diode Barrier
9.3.7.3 3D Modeling of the Extrinsic Structure of the Diodes
9.3.7.4 Modeling and Optimization of the Diode Cell
9.3.7.5 Input and Output Matching Circuits
9.4 Technology of THz Diode Frequency Multipliers
9.4.1 From Whisker‐Contacted Diodes to Planar Discrete Diodes
9.4.2 Semi‐Monolithic Frequency Multipliers at THz Frequencies
9.4.3 THz Local Oscillators for the Heterodyne Instrument of Herschel Space Observatory
9.4.4 First 2.7 THz Multiplier Chain with More Than 10 μW of Power at Room Temperature
9.4.5 High Power 1.6 THz Frequency Multiplied Source for Future 4.75 THz Local Oscillator
9.5 Power‐Combining at Sub‐Millimeter Wavelength
9.5.1 In‐Phase Power Combining
9.5.1.1 First In‐Phase Power‐Combined Submillimeter‐Wave Frequency Multiplier
9.5.1.2 In‐Phase Power Combining at 900 GHz
9.5.1.3 In‐Phase Power‐Combined Balanced Doublers
9.5.2 In‐Channel Power Combining
9.5.3 Advanced on‐Chip Power Combining
9.5.3.1 High Power 490–560 GHz Frequency Tripler
9.5.3.2 Dual‐Output 550 GHz Frequency Tripler
9.5.3.3 High‐Power Quad‐channel 165–195 GHz Frequency Doubler
9.6 Conclusions and Perspectives
Exercises
References
Explanatory Notes (see superscripts in text)
10 GaN Multipliers
10.1 Introduction. 10.1.1 Frequency Multipliers
10.1.2 Properties of Nitride Materials
10.1.3 Motivation and Challenges
10.2 Theoretical Considerations of GaN Schottky Diode Design
10.2.1 Analysis by Analytical Equations. 10.2.1.1 Nonlinearity and Harmonic Generation
10.2.1.2 Nonlinearity of Ideal Schottky Diode
10.2.1.3 Series Resistance
10.2.2 Analysis by Numeric Simulation
10.2.2.1 Introduction of Semiconductor Device Numerical Simulation
10.2.2.2 Parameters for GaN‐Based Device Simulation
10.2.2.3 Simulation Results. 10.2.2.3.1 Device Structure
10.2.2.3.2 Breakdown Voltage
10.2.2.3.3 I–V Characteristics
10.2.2.3.4 Series Resistance
10.2.2.3.5 C–V Characteristics
10.2.2.3.6 Time‐domain Transient Analysis
10.2.3 Conclusions on Theoretical Considerations of GaN Schottky Diode Design
10.3 Fabrication Process of GaN Schottky Diodes
10.3.1 Fabrication Process
10.3.2 Etching
10.3.3 Metallization. 10.3.3.1 Ohmic Contacts on GaN
10.3.3.2 Schottky Contacts on GaN
10.3.3.2.1 Analysis of Schottky Contact Characteristics
10.3.3.2.2 Oxygen Plasma Before Schottky Metallization
10.3.4 Bridge Interconnects
10.3.4.1 Dielectric Bridge
10.3.4.2 Optical Air‐bridge
10.3.4.3 E‐beam Air‐bridge
10.3.5 Conclusion on Fabrication Process of GaN Schottky Diodes
10.4 Small‐signal High‐frequency Characterization of GaN Schottky Diodes
10.4.1 Current‐voltage Characteristics
10.4.2 Small‐signal Characterization and Equivalent Circuit Modeling
10.4.2.1 Step 1. Parasitic Elements
10.4.2.2 Step 2. Junction Capacitance
10.4.2.3 Step 3. Optimization
10.4.2.4 Summary
10.4.3 Results
10.4.4 Conclusion
10.5 Large‐signal On‐wafer Characterization
10.5.1 Characterization Approach
10.5.2 Large Signal Measurements of GaN Schottky Diodes. 10.5.2.1 LSNA With 50 Ω Load
10.5.2.2 Time Domain Waveforms
10.5.2.3 Instant C–V Under Large‐signal Driven Conditions
10.5.2.4 Power Handling Characteristics
10.5.3 LSNA With Harmonic Load‐pull
10.5.4 Conclusion
10.6 GaN Diode Implementation for Signal Generation
10.6.1 Large‐signal Modeling of GaN Schottky Diodes
10.6.2 Frequency Doubler
10.7 Multiplier Considerations for Optimum Performance
Exercises
References
11 THz Resonant Tunneling Devices
11.1 Introduction
11.2 Principle of RTD Oscillators
11.2.1 Basic Operation of RTD
11.2.2 Principle of Oscillation
11.2.3 Effect of Electron Delay Time
11.2.3.1 Degradation of NDC at High Frequency
11.2.3.2 Generation of Reactance at High Frequency
11.3 Structure and Oscillation Characteristics of Fabricated RTD Oscillators
11.3.1 Actual Structure of RTD Oscillators
11.3.2 High‐frequency Oscillation
11.3.3 High‐output Power Oscillation
11.4 Control of Oscillation Spectrum and Frequency
11.4.1 Oscillation Spectrum and Phase‐Locked Loop
11.4.2 Frequency‐tunable Oscillators
11.5 Targeted Applications. 11.5.1 High‐speed Wireless Communications
11.5.2 Spectroscopy
11.5.3 Other Applications and Expected Future Development
Exercises
References
12 Wireless Communications in the THz Range
12.1 Introduction
12.2 Evolution of Telecoms Toward THz. 12.2.1 Brief Historic
12.2.2 Data Rate Evolution
12.2.3 THz Waves: Propagation, Advantages, and Disadvantages
12.2.4 Frequency Bands
12.2.5 Potential Scenarios
12.2.6 Comparison Between FSO and THz
12.3 THz Technologies: Transmitters, Receivers, and Basic Architecture. 12.3.1 THz Sources
12.3.2 THz Receivers
12.3.3 Basic Architecture of the Transmission System
12.4 Devices/Function Examples for T‐Ray CMOS. 12.4.1 Photomixing Techniques for THz CMOS
12.4.2 THz Modulated Signals Enabled by Photomixing
12.4.3 Other Techniques for the Generation of Modulated THz Signals
12.4.4 Integration, Interconnections, and Antennas. 12.4.4.1 Integration
12.4.4.2 Antennas
12.5 THz Links. 12.5.1 Modulations and Key Indicators of a THz Communication Link
12.5.2 State‐of‐the‐Art of THz Links. 12.5.2.1 First Systems
12.5.2.2 Photonics‐Based Demos
12.5.2.3 Electronic‐Based Demos
12.5.2.4 Beyond 100 GHz High Power Amplification
12.5.2.5 Table of Reported Systems
12.6 Toward Normalization of 100G Links in the THz Range
12.7 Conclusion
12.8 Acronyms
E12.1 Link Budget of a THz Link
References
13 THz Applications: Devices to Space System
13.1 Introduction
13.1.1 Why Is THz Technology Important for Space Science?
13.1.2 Fundamentals of THz Spectroscopy
13.1.3 THz Technology for Space Exploration
13.2 THz Heterodyne Receivers
13.2.1 Local Oscillators
13.2.1.1 Frequency Multiplied Chains
13.2.2 Mixers. 13.2.2.1 Room Temperature Schottky Diode Mixers
13.2.2.2 SIS Mixer Technology
13.2.2.3 Hot Electron Bolometric (HEB) Mixers
13.2.2.4 State‐of‐the‐Art Receiver Sensitivities
13.3 THz Space Applications
13.3.1 Planetary Science: The Case for Miniaturization
13.3.2 Astrophysics: The Case for THz Array Receivers
13.3.3 Earth Science: The Case for Active THz Systems
13.4 Summary and Future Trends
Acknowledgment
Exercises
References
Index. a
b
c
d
e
f
g
h
i
j
k
l
m
n
o
p
q
r
s
t
u
v
w
WILEY END USER LICENSE AGREEMENT
Отрывок из книги
Edited by
.....
by substituting (2.46) and (2.47) in (2.45), the amplitude of the field at equivalent aperture of the lens is related to the amplitude of the incident field as:
(2.48)
.....