Catalytic Asymmetric Synthesis
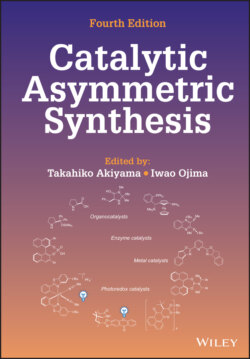
Реклама. ООО «ЛитРес», ИНН: 7719571260.
Оглавление
Группа авторов. Catalytic Asymmetric Synthesis
Table of Contents
List of Tables
List of Illustrations
Guide
Pages
CATALYTIC ASYMMETRIC SYNTHESIS
PREFACE
PREFACE TO THE FIRST EDITION
LIST OF CONTRIBUTORS
1 ASYMMETRIC ENAMINE AND IMINIUM ION CATALYSIS
1.1. INTRODUCTION
1.2. REPRESENTATIVE ORGANOCATALYSTS. 1.2.1. Introduction
1.2.2. Reactivity of Diphenylprolinol Silyl Ether Catalyst and MacMillan’s Catalyst
1.2.3. Cinchona Amine‐Based Catalysts
1.3. ENAMINE. 1.3.1. Aldol Reaction
1.3.2. Mannich Reaction
1.3.3. Other Functionalization of the α‐Position of Carbonyl Groups
1.3.4. Michael Reaction
1.3.5. Dienamine and Trienamine as an Intermediate [33]
1.4. IMINIUM ION. 1.4.1. Introduction of an Iminium Ion
1.4.2. Two Reaction Paths
1.4.3. Diels‐Alder Type Reaction
1.4.4. Michael Reaction
1.5. DOMINO REACTION. 1.5.1. Introduction of Domino (Cascade and Tandem) Reactions
1.5.2. Enders’ Work
1.6. DOMINO REACTION AND TOTAL SYNTHESIS
1.6.1. Steroid Skeleton
1.6.2. α‐Skytanthine and Quinine
1.6.3. (+)‐Lycoposerramine Z
1.6.4. Estradiol Methyl Ether
1.6.5. MacMillan’s Alkaloid Synthesis
1.6.6. Prostaglandin E1 Methyl Ester
1.6.7. Corey Lactone
1.7. COMBINATION OF TWO CATALYSTS
1.7.1. Combination of Two Organocatalysts
1.7.2. Combination of Organocatalyst and Metal Catalyst
1.7.2.1. Enamine and Metal Catalyst
1.7.2.2. Iminium Ion and Transition Metal Catalyst
1.7.3. Two Chiral Catalysts
1.8. CONCLUSION
REFERENCES
2 ASYMMETRIC ACID ORGANOCATALYSIS
2.1. INTRODUCTION
2.2. FEATURES OF CHIRAL BRØNSTED ACIDS
2.2.1. Acidity of Chiral Brønsted Acids
2.2.2. Mode of Activation of Chiral Phosphoric Acids and Related Compounds
2.2.3. Effect of Metal Salts
2.3. NUCLEOPHILIC REACTIONS. 2.3.1. Reactions with Imines and Iminium Salts
2.3.2. Reactions with Carbonyl Compounds and Oxonium Salts
2.4. CYCLOADDITION REACTIONS. 2.4.1. Diels‐Alder Reactions
2.4.2. Aza‐Diels‐Alder Reactions
2.4.3. Oxa‐Diels‐Alder Reactions
2.4.4. Other Cycloaddition Reactions
2.4.5. Nazarov Cyclizations
2.5. MICHAEL REACTIONS
2.6. REDUCTION. 2.6.1. Reduction of Imines. 2.6.1.1. Transfer Hydrogenation of Ketimines
2.6.2. Reduction of Ketones
2.6.3. Reduction of Alkenes
2.7. ADDITION TO ALKENES
2.8. SUBSTITUTION REACTIONS
2.9. REARRANGEMENT REACTIONS
2.10. MISCELLANEOUS REACTIONS
2.11. CONSTRUCTION OF AXIALLY, PLANAR, AND HELICALLY CHIRAL COMPOUNDS
2.12. COMBINATION WITH TRANSITION METAL CATALYSTS [25–27]
2.13. COMBINATION WITH PHOTOREDOX CATALYST
2.14. CONCLUSION
ACKNOWLEDGMENTS
REFERENCES
3 ASYMMETRIC BASE ORGANOCATALYSIS
3.1. INTRODUCTION
3.2. CHIRAL TERTIARY AMINE CATALYSTS: CHIRAL ACID–BASE BIFUNCTIONAL CATALYSIS
3.2.1. Application of Designed Pronucleophiles
3.2.2. Carbon‐Heteroatom Bond Formations
3.2.3. Other Applications
3.3. CHIRAL GUANIDINE CATALYSTS
3.4. OTHER CHIRAL UNCHARGED ORGANOBASE CATALYSTS: CHIRAL ORGANOSUPERBASES
3.4.1. Chiral Cyclopropenimine Catalysts
3.4.2. Chiral Triaryliminophosphorane Catalysts
3.4.3. Chiral P1‐Phosphazene Catalysts
3.4.4. Chiral Higher‐Order Phosphazene Catalysts
3.5. CONCLUSION AND OUTLOOK
REFERENCES
4 ASYMMETRIC PHASE‐TRANSFER AND ION‐PAIR ORGANOCATALYSES
4.1. INTRODUCTION
4.2. CHIRAL CATION. 4.2.1. Chiral Cation Phase‐Transfer Catalysis. 4.2.1.1. Alkylation
4.2.1.2. Addition to Michael Acceptors
4.2.1.3. Addition to Carbonyls and Imines
4.2.1.4. Arylation
4.2.1.5. Carbon‐Heteroatom (C‐X) Bond Formation
4.2.2. Transition‐Metal/Chiral Cation Dual Catalysis
4.2.3. Cation‐Binding Catalysis
4.3. CHIRAL‐ANION
4.3.1. Iminium
4.3.2. Oxocarbenium
4.3.3. Carbocation
4.3.4. Miscellaneous
4.3.5. Chiral‐Anion Phase Transfer. 4.3.5.1. Halogenation
4.3.5.2. Amination
4.3.5.3. Miscellaneous Transformations
4.3.6. Transition‐Metal/Chiral‐Anion Dual Catalysis
4.3.7. Anion‐Binding Catalysis
4.3.7.1. Nonaromatic Cations
4.3.7.2. Cationic Heterocycles
4.3.7.3. Asymmetric Ring Opening of Strained Heterocycles
4.4. CONCLUSION
REFERENCES
5 ASYMMETRIC PEPTIDE CATALYSIS
5.1 INTRODUCTION
5.2 CATALYSIS BY N‐TERMINAL AMINO GROUP OF PEPTIDES
5.2.1 Enamine Catalysis
5.2.2 Iminium Ion Catalysis
5.2.3 Other Type of Peptide Catalysts That Utilize Terminal Amino Groups
5.3 CATALYSIS BY SIDE CHAIN FUNCTIONAL GROUP ON PEPTIDES
5.3.1 Histidine‐Based Peptide Catalysis
5.3.2 Aspartate/Glutamate‐Based Peptide Catalysis
5.3.3 Arg/Lys‐Based Peptide Catalysis
5.3.4 Cysteine‐Based Peptide Catalysis
5.4 CATALYSIS BY FUNCTIONAL GROUPS COVALENTLY BOUND TO PEPTIDES
5.4.1 Peptide Catalysts That Have Catalytic Center Connected to N‐Terminal
5.4.2. Peptide Catalysts That Have Catalytic Center on Side Chain of Amino Acid
5.5 PEPTIDE CATALYSIS WITH OTHER TYPES OF CATALYTIC CENTERS
5.6 CONCLUSION
REFERENCES
6 ASYMMETRIC CARBENE CATALYSIS: A BRIEF HIGHLIGHT OF DEVELOPMENTS IN THE PAST DECADE
6.1. EARLY DEVELOPMENT OF ASYMMETRIC NHC CATALYSIS
6.1.1. Early Discoveries on NHC‐Mediated Reactions: Benzoin and Related Reactions via Acyl Anion Intermediates
6.1.2. Moving from Simple Aldehydes to Enals and α‐Functionalized Aldehydes: A Key Progress During the First Decade of This Century. 6.1.2.1. Activation of Enals via Homoenolate Intermediates
6.1.2.2. Functionalization of Enals via Enolate Intermediates
6.1.2.3. Oxidation of Homoenolate to α,β‐Unsaturated Acyl Azolium Intermediates
6.1.2.4. Activation of α‐Functionalized Aldehydes for Asymmetric Reactions
6.1.3. Remaining Challenges When the Last Decade Started
6.2. ACTIVATION OF SUBSTRATES BEYOND ALDEHYDES. 6.2.1. Activations of Stable Carboxylic Esters. 6.2.1.1. α‐Carbon Activation of Carboxylic Esters
6.2.1.2. β‐sp2‐Carbon Activation of α,β‐Unsaturated Carboxylic Esters
6.2.1.3. γ‐Carbon Activation of α,β‐Unsaturated Carboxylic Esters
6.2.1.4. β‐sp3‐Carbon Activation of Saturated Esters
6.2.2. Activation of Ketenes
6.2.3. Activation of Imines
6.2.4. Activation of Other Substrates
6.3. SINGLE‐ELECTRON TRANSFER ACTIVATION AND RADICAL REACTIONS. 6.3.1. Oxidation of Aldehydes to Esters
6.3.2. Reductive Coupling Reactions Involving Nitroalkenes and Nitrobenzyl Bromides
6.3.3. Activation of Enal on β‐Carbon via SET for Asymmetric Reactions
6.3.4. Radical–Radical Coupling via NHC‐Catalyzed Activation of Aldehydes
6.3.5. Visible‐Light‐Driven Radical Reactions
6.4. NHC AS NON‐COVALENT (BRØNSTED BASE) CATALYSTS
6.5. COOPERATIVE CATALYSIS OF NHCs WITH OTHER CATALYSTS. 6.5.1. Dual Catalysis of NHC Organocatalysts and Transition Metal Catalysts
6.5.1.1. NHC and Pd
6.5.1.2. NHC and Cu
6.5.1.3. NHC and Au
6.5.1.4. NHC and Ir
6.5.1.5. Other Important Studies
6.5.2. Dual Catalysis of NHC Organocatalysts and Lewis Acid Co‐catalysts/Additives
6.5.3. Dual Catalysis of NHC Organocatalysts and Brønsted Acids
6.5.4. Dual Catalysis of NHC Organocatalysts and Other Catalysts
6.6. SYNTHETIC APPLICATIONS OF NHC CATALYSIS. 6.6.1. Kinetic Resolution and Desymmetrization
6.6.1.1. NHC‐Catalyzed Kinetic Resolutions
6.6.1.2. NHC‐Catalyzed Dynamic Kinetic Resolutions
6.6.1.3. NHC‐Catalyzed Desymmetrizations
6.6.2. NHC Catalysis in Natural Product Synthesis
6.7. SUMMARY AND OUTLOOK
REFERENCES
7 ASYMMETRIC HYPERVALENT IODINE CATALYSIS
7.1 INTRODUCTION
7.2 OXIDATIVE DEAROMATIVE COUPLING OF ARENOLS
7.3 OXIDATIVE α‐FUNCTIONALIZATION OF CARBONYL COMPOUNDS
7.4 OXIDATIVE DIFUNCTIONALIZAITON OF ALKENES
7.5 CONCLUSION AND OUTLOOK
REFERENCES
8 ASYMMETRIC VISIBLE‐LIGHT PHOTOREDOX CATALYSIS
8.1. INTRODUCTION
8.2. DUAL CATALYSIS APPROACH. 8.2.1. Lewis Base Catalysis. 8.2.1.1. Enamine Catalysis. 8.2.1.1.1. SET Reduction of Electrophilic Radical Precursors
8.2.1.1.2. SET Oxidation of Chiral Enamine
8.2.1.1.3. SET Reduction of Electrophilic Radical Precursor and Oxidation of Chiral Enamine
8.2.1.1.4. α‐Oxygenation
8.2.1.2. Iminium Catalysis
8.2.2. Hydrogen‐Bonding Catalysis
8.2.3. Brønsted Base Catalysis
8.2.4. Brønsted Acid Catalysis
8.2.5. Lewis Acid Catalysis
8.2.5.1. Activation of Substrates toward Radical Addition
8.2.5.2. Activation of Electron‐Deficient Organic Substrates toward Photocatalytic Reduction
8.2.5.3. Chiral Enolate Complex as Reductive Quencher and Acceptor of Electrophilic Radicals
8.2.6. Phase‐Transfer Catalysis
8.3. SINGLE BIFUNCTIONAL CATALYST APPROACH. 8.3.1. Chiral Organophotocatalysts. 8.3.1.1. Enamine Catalysis
8.3.1.2. Ion‐pair Catalysis
8.3.1.3. Chiral Brønsted Acid Catalysis
8.3.2. Chiral Organometallic Photocatalysts
8.3.2.1. Chiral‐at‐Metal Photocatalysts
8.3.2.2. Chiral Ligands and Photocatalysts
8.4. CONCLUSION
REFERENCES
9 ASYMMETRIC PHOTOREDOX REACTIONS WITHOUT PHOTOCATALYSTS
9.1. GENERAL INTRODUCTION
9.2 PHOTOEXCITATION OF ORGANOCATALYTIC INTERMEDIATES
9.2.1 Enamine Catalysis in EDA Complex Photoactivation
9.2.2 Phase Transfer Catalysis in EDA Complex Photoactivation
9.2.3 Iminium Ion Catalysis in EDA Complex Photoactivation
9.2.4 Direct Photoexcitation of Enamines
9.2.5 Direct Photoexcitation of Iminium Ions
9.3 PHOTOEXCITATION OF METAL‐BASED INTERMEDIATES
9.3.1 Use of Chiral Lewis Acids to Form Photoactive Intermediates
9.3.2 Photoexcitation of Organometallic Intermediates
9.4 PHOTOCHEMISTRY AND BIOCATALYSIS
9.4.1 EDA Complex Photochemistry and Enzymatic Catalysis
9.4.2 Direct Photoexcitation Strategies in Enzymatic Catalysis
9.5 METHODS BASED ON THE DIRECT EXCITATION OF SUBSTRATES
9.6 CONCLUSIONS
ACKNOWLEDGMENTS
REFERENCES
10 ENANTIOSELECTIVE PHOTOCHEMICAL [2+2] CYCLOADDITION REACTIONS
10.1. INTRODUCTION
10.2. CHIRAL ORGANOCATALYSTS
10.2.1. Xanthone and Thioxanthone
10.2.2. Thioureas
10.2.3. Brønsted Acids
10.2.4. Iminium Ions
10.3. CHIRAL METAL CATALYSTS
10.3.1. Transition Metals and Lanthanides
10.3.2. AlBr3‐Activated Oxazaborolidines
10.4. DUAL CATALYSIS
10.4.1. Electron Transfer
10.4.2. Energy Transfer. 10.4.2.1. Lewis Acid Catalysis
10.4.2.2. Eniminium Ions
10.5. CHIRAL METAL‐ORGANIC CAGES
10.6. CONCLUDING REMARKS
ACKNOWLEDGMENTS
REFERENCES
11 ASYMMETRIC C–H FUNCTIONALIZATION OF C(sp2)–H BOND
11.1. INTRODUCTION
11.2. PALLADIUM CATALYSIS
11.2.1. Phosphorus‐Based Ligands
11.2.2. Monoprotected Amino Acids as Chiral Ligands
11.2.3. Other Ligands
11.2.4. Chiral Transient Auxiliary
11.2.5. Chiral Auxiliaries
11.2.6. Cooperative Catalysis
11.2.7. Electrochemical C−H Activations
11.3. RHODIUM CATALYSIS. 11.3.1. Chiral Cpx‐Based Catalysts
11.3.2. In‐Situ Generated Chiral Complexes
11.3.2.1. Phosphine Ligand
11.3.3. Other Strategies
11.4. IRIDIUM CATALYSIS
11.4.1. C–C Bond Formations. 11.4.1.1. Phosphorus‐Based Ligands
11.4.1.2. Chiral Diene Ligand
11.4.2. C−H Borylations
11.4.3. C−H Silylations
11.5. RUTHENIUM CATALYSIS
11.5.1. Chiral Amine as the TDG
11.5.2. Chiral Acid
11.6. SCANDIUM CATALYSIS
11.7. NICKEL CATALYSIS
11.7.1. Formyl C–H Activation
11.7.2. Intramolecular Reactions
11.7.3. Intermolecular Reactions
11.8. COBALT CATALYSIS
11.8.1. Cobalt Catalysis under Reducing Conditions
11.8.2. Cobalt(III) Complexes. 11.8.2.1. Chiral Acid
11.8.2.2. Chiral Cyclopentadienyl Cobalt Complex
11.9. COPPER CATALYSIS
11.10. IRON CATALYSIS
11.10.1. Phosphine‐Based Ligands
11.10.2. N‐Heterocyclic Carbene
11.11. CONCLUSIONS
ACKNOWLEDGMENTS
REFERENCES
12 ASYMMETRIC C–H FUNCTIONALIZATION OF C(sp3)–H BOND
12.1. INTRODUCTION
12.2. C(sp3)–H BOND INSERTION BY METAL CARBENOIDS AND METAL NITRENOIDS
12.2.1. C(sp3)–H Bond Insertion of Metal Carbenoids. 12.2.1.1. Introduction
12.2.1.2. Intermolecular C(sp3)–H Functionalization
12.2.1.2.1. Insertion into Unactivated C(sp3)–H Bonds
12.2.1.2.2. Insertion into Allylic and Benzylic C(sp3)–H Bonds
12.2.1.2.3. Insertion into C(sp3)–H Bonds α to Heteroatoms
12.2.1.2.4. Insertion into C(sp3)–H Bonds β to Silicon
12.2.1.2.5. Combined C(sp3)–H Functionalization/Cope Rearrangement
12.2.1.3. Intramolecular C(sp3)–H Functionalization
12.2.2. C(sp3)–H Bond Insertion of Metal Nitrenoids. 12.2.2.1. Introduction
12.2.2.2. C(sp3)–H Functionalization with Iminoiodinanes. 12.2.2.2.1. Intermolecular C(sp3)–H Amination with Iminoiodinanes
12.2.2.2.2. Intramolecular C(sp3)–H Amination with Iminoiodinanes
12.2.2.3. C(sp3)–H Functionalization with Azides. 12.2.2.3.1. Intermolecular C(sp3)–H Amination with Azides
12.2.2.3.2. Intramolecular C(sp3)–H Amination with Azides
12.2.2.4. C(sp3)–H Functionalization with N‐(Sulfonyloxy)Carbamates
12.2.2.5. C(sp3)–H Functionalization with Dioxazolones
12.2.2.6. C(sp3)–H Functionalization with Hydroxylamine Derivatives
12.3. CONCERTED METALATION‐DEPROTONATION FOR ASYMMETRIC C(sp3)–H ACTIVATION
12.3.1. Pd(0)‐catalyzed C(sp3)–H Activation
12.3.1.1. Pd(0)‐catalyzed Enantioselective C(sp3)–C(sp2) Bond Formation
12.3.1.2. Pd(0)‐catalyzed C(sp3)–C(sp3) Bond Formation
12.3.2. Pd(II)‐catalyzed C(sp3)–H Activation
12.3.2.1. Pd(II)/Pd(0)‐catalyzed C(sp3)–H Activation
12.3.2.2. Pd(II)/Pd(IV)‐catalyzed C(sp3)–H Activation
12.3.3. Pd(II)‐catalyzed Allylic C(sp3)–H Activation
12.3.4. Other Transition Metal Catalyzed Asymmetric C(sp3)–H Activation
12.4. C(sp3)–H ACTIVATION VIA OXIDATIVE ADDITION MECHANISM. 12.4.1. Introduction
12.4.2. C–C Bond Forming Reaction. 12.4.2.1. Allylic and Benzylic C–H Bond Activation
12.4.2.2. α‐Heteroatom C–H Bond Activation
12.4.3. C(sp3)–H Borylation
12.4.4. C(sp3)–H Silylation
12.4.4.1. Rhodium‐catalyzed Silylation
12.4.4.2. Iridium‐catalyzed Silylation
12.5. CONCLUSION
REFERENCES
13 ASYMMETRIC CARBON–HALOGEN BOND FORMING REACTIONS (EXCLUDING C–H ACTIVATION PROCESSES)
13.1. INTRODUCTION
13.2. ASYMMETRIC C–F BOND FORMATION WITH METAL CATALYSTS
13.2.1. Allylic Substitution
13.2.2. Addition to Alkenes
13.2.3. Lewis Acid Catalysis
13.3. ASYMMETRIC C–F BOND FORMING REACTIONS USING CHIRAL PHASE TRANSFER CATALYSIS
13.3.1. α‐Fluorination of Simple Oxo Compounds and Their Derivatives
13.3.2. α‐Fluorination of β‐Ketoesters
13.3.3. Transformations of Olefin Bonds
13.3.4. Dearomative Fluorination of Arenes
13.3.5. Application of Neutral Phase Transfer Catalysts
13.4. ASYMMETRIC ORGANOCATALYTIC FORMATION OF C–F BONDS
13.4.1. α‐Fluorination of Aldehydes
13.4.2. α‐Fluorination of Ketones
13.4.3. α‐Fluorination of β‐Oxo Carbonyl Compounds
13.4.4. α‐Fluorination of Simple Carboxylic Acid Derivatives
13.4.5. Fluorinative Transformations of Carbon–Carbon Double Bonds
13.5. ASYMMETRIC FORMATION OF C–F BONDS VIA MISCELLANEOUS REACTIONS. 13.5.1. Fluorination Using N‐Heterocyclic Carbene Catalysis
13.5.2. Fluorination Using Chiral Iodoarene Difluorides
13.6. ENANTIOSELECTIVE CHLORINATION
13.6.1. Organocatalysis. 13.6.1.1. Chlorination of Carbonyl Compounds
13.6.1.2. Dichlorination of Alkenes
13.6.1.3. Chlorofunctionalization of Alkenes
13.6.2. Strategies Based on Chiral Metal Complexes. 13.6.2.1. Chlorination of β‐Ketoesters
13.6.2.2. Chlorination of Alkenes
13.7. ENANTIOSELECTIVE BROMINATION
13.7.1. Strategies Based on Chiral Metal Complexes
13.7.1.1. Bromination of Alkenes
13.7.1.2. Bromoaminocyclization Reactions
13.7.2. Strategies Based on the Use of Chiral Auxiliaries. 13.7.2.1. Bromolactonization Reactions
13.7.3. Strategies Based on the Use of Organocatalysts. 13.7.3.1. Bromocyclization Reactions
13.7.3.2. Bromofunctinalization of Carbonyl Compounds
13.8. ASYMMETRIC CARBON–IODINE BOND FORMATION
13.8.1. Iodoaminations
13.8.2. Iodolactonizations
13.9. CONCLUSIONS
ACKNOWLEDGMENTS
REFERENCES
14 ENZYME‐CATALYZED ASYMMETRIC SYNTHESIS
14.1. TYPES OF BIOCATALYZED PROCESSES
14.2. KINETIC RESOLUTION
14.3. DYNAMIC PROCESSES IN BIOCATALYZED ASYMMETRIC SYNTHESIS
14.4. DERACEMIZATIONS
14.5. PARALLEL KINETIC RESOLUTIONS
14.6. DESYMMETRIZATION
14.7. MULTI(CHEMO)ENZYMATIC REACTIONS
14.8. CONCLUSIONS AND OUTLOOK
REFERENCES
15 ASYMMETRIC HYDROGENATION
15.1. INTRODUCTION
15.2. ASYMMETRIC HYDROGENATION OF FUNCTIONALIZED OLEFINS. 15.2.1. Introduction
15.2.2. Rhodium Catalysts
15.2.3. Cobalt and Nickel Catalysts
15.2.4. Iridium Catalysts
15.2.5. Ruthenium Catalysts
15.3. ASYMMETRIC HYDROGENATION OF UNFUNCTIONALIZED OLEFINS
15.3.1. Iridium‐Catalyzed Hydrogenations
15.3.1.1. Model Substrate: (E)‐2‐Methyl‐2‐Stilbene
15.3.1.2. Trisubstituted Alkenes and Dienes
15.3.1.3. Terminal (1,1,‐Disubstituted) Alkenes
15.3.1.4. Tetrasubstituted Alkenes
15.3.2. Rhodium‐Catalyzed Hydrogenations
15.3.3. Cobalt‐Catalyzed Hydrogenations
15.4. ASYMMETRIC HYDROGENATION OF KETONES
15.4.1. Ruthenium Catalysts. 15.4.1.1. Diphosphine‐Diamine Catalytic Systems
15.4.1.2. Arene‐Diamine Catalytic Systems
15.4.1.3. Other Ruthenium Catalytic Systems
15.4.2. Rhodium Catalysts
15.4.3. Iridium Catalysts
15.4.4. Iron Catalysts
15.4.5. Manganese and Cobalt Catalysts
15.5. ASYMMETRIC HYDROGENATION OF IMINES
15.5.1. Heterocyclic Imines and Nitrogenated Heteroaromatics
15.5.2. Acyclic Imines
15.5.3. Reductive Amination
15.6. CONCLUSIONS
REFERENCES
16 ASYMMETRIC NUCLEOPHILIC ADDITION TO KETONES AND KETIMINES AND CONJUGATE ADDITION REACTIONS
16.1. INTRODUCTION
16.2. ASYMMETRIC NUCLEOPHILIC ADDITION TO KETONES
16.2.1. Asymmetric Nucleophilic Addition of Grignard Reagents to Ketones
16.2.2. Asymmetric Nucleophilic Addition of Organoboron Reagents to Ketones
16.2.2.1. Arylation Reactions
16.2.2.2. Allylation Reactions
16.2.2.3. Propargylation Reactions
16.2.3. Asymmetric Nucleophilic Addition of Organotitanium Reagents to Ketones
16.3. ASYMMETRIC NUCLEOPHILIC ADDITION TO KETIMINES
16.3.1. Asymmetric Nucleophilic Addition of Grignard Reagents to Ketimines
16.3.2. Asymmetric Nucleophilic Addition of Organoboron Reagents to Ketimines
16.3.2.1. Rh‐Catalyzed Enantioselective Additions of Organoboron Reagents to Ketimines
16.3.2.2. Pd‐Catalyzed Enantioselective Additions of Organoboron Reagents to Ketimines
16.3.2.3. Other Transition‐Metal‐Catalyzed Enantioselective Additions of Organoboron Reagents to Ketimines
16.4. CATALYTIC ASYMMETRIC CONJUGATE ADDITION
16.4.1. Asymmetric Conjugate Addition (ACA) of Grignard Reagents
16.4.1.1. ACA to Alkenyl‐Heteroarenes
16.4.1.2. ACA to α,β‐Unsaturated Carboxamides and Carboxylic Acids
16.4.1.3. ACA to Heterocyclic Michael Acceptors
16.4.2. Asymmetric Conjugate Addition of Organozinc Reagents
16.4.2.1. ACA to Cyclic Enones
16.4.2.2. ACA to Acyclic Enones
16.4.2.3. ACA to Nitroalkenes
16.4.3. Asymmetric Conjugate Addition of Organozirconium Reagents
16.4.4. Asymmetric Conjugate Addition of Organoborane Reagents
16.4.4.1. ACA to α,β‐Unsaturated Ketimines
16.4.4.2. ACA to α,β‐Unsaturated Enones
16.4.4.3. ACA to Nitroalkenes
16.4.4.4. ACA to α,β‐Unsaturated Esters
16.4.4.5. ACA to Other Michael Acceptors
16.5. CONCLUSIONS
ACKNOWLEDGMENTS
REFERENCES
17 ASYMMETRIC ALLYLIC ALKYLATION, ALLYLATION, AND RELATED REACTIONS
17.1. INTRODUCTION AND SCOPE
17.2. PALLADIUM‐CATALYZED ENANTIOSELECTIVE ALLYLIC ALKYLATIONS
17.2.1. Pd‐Catalyzed Asymmetric Allylic and Benzylic Substitution with Stabilized Carbon and Heteroaromatic Nucleophiles
17.2.1.1. Monodentate P‐ligands
17.2.1.2. C2‐Symmetric P,P‐ligands
17.2.1.3. Bidentate P,N(sp2)‐ligands
17.2.2. Pd‐Catalyzed Asymmetric Allylic Substitution with Non‐Stabilized C‐nucleophiles
17.2.3. Pd‐Catalyzed Decarboxylative Allylic Substitution
17.2.4. Pd‐Catalyzed Asymmetric Oxidative Allylic Alkylation
17.3. NICKEL‐ AND PLATINUM‐CATALYZED ALLYLIC ALKYLATION. 17.3.1. Ni‐Catalyzed Allylic Alkylation
17.3.2. Pt‐Catalyzed Allylic Alkylation
17.4. MOLYBDENUM‐ AND TUNGSTEN‐CATALYZED ENANTIOSELECTIVE ALLYLIC ALKYLATION. 17.4.1. Mb‐Catalyzed Allylic Alkylation
17.4.2. W‐Catalyzed Allylic Alkylation
17.5. IRON‐ AND RUTHENIUM‐CATALYZED ALLYLIC ALKYLATION. 17.5.1. Fe‐Catalyzed Allylic Alkylation
17.5.2. Ru‐Catalyzed Allylic Alkylation
17.6. RHODIUM‐CATALYZED ENANTIOSELECTIVE ALLYLIC ALKYLATION
17.7. IRIDIUM‐CATALYZED ENANTIOSELECTIVE ALLYLIC ALKYLATION
17.7.1. Ir‐Catalyzed Allylic Alkylation with Stabilized Nucleophiles
17.7.2. Ir‐Catalyzed Allylic Alkylation with Non‐Stabilized Nucleophiles
17.7.3. Ir‐Catalyzed Allylic Alkylation with Enamine Catalysis
17.7.4. Ir‐Catalyzed Allylic Alkylation with Arene Nucleophiles
17.7.5. Ir‐Catalyzed Allylic Alkylation with Olefin Nucleophiles
17.7.6. Ir‐Catalyzed Allylic Alkylation with Organometallic Nucleophiles
17.7.7. Ir‐Catalyzed Allylic Alkylation with Umpolung Reactivity of Imines
17.8. COPPER‐CATALYZED ENANTIOSELECTIVE ALLYLIC ALKYLATION
17.8.1. Allylic Alkylation of Grignard Reagents
17.8.2. Allylic Alkylation of Organolithium Reagents
17.8.3. Allylic Alkylation of Organoaluminum Reagents
17.8.4. Allylic Alkylation of Organoboron Reagents
17.8.5. Allylic Alkylation of Organozirconium Reagents
17.8.6. Other Carbon Nucleophiles
17.9. COBALT‐CATALYZED ENANTIOSELECTIVE ALLYLIC ALKYLATION
17.10. CONCLUDING REMARKS
REFERENCES
18 ASYMMETRIC CARBOMETALLATIONS INCLUDING CARBOCYCLIZATIONS
18.1. INTRODUCTION
18.2. CARBOMETALLATIONS WITH MAIN GROUP METALS
18.2.1. Carboaluminations
18.2.2. Carbomagnesiations and Carbozincations
18.3. CARBOMETALLATIONS WITH TRANSITION METALS
18.3.1. Carbopalladations
18.3.2. Carbonickelations
18.3.3. Carborhodations
18.3.4. Carbocobaltations
18.4. CONCLUSION
REFERENCES
19 ASYMMETRIC SYNTHESIS OF AXIALLY CHIRAL COMPOUNDS
19.1. INTRODUCTION
19.2. METAL CATALYSIS. 19.2.1. Biaryl Atropisomers. 19.2.1.1. Formation of C(aryl)‐C(aryl) Bond. 19.2.1.1.1. Transition Metal‐Catalyzed Cross‐Coupling
19.2.1.1.2. Direct C‐H Arylation
19.2.1.1.3. Dehydrogenative Cross‐Coupling
19.2.1.2. De Novo Arene Formation
19.2.1.3. Atroposelective Functionalization of Prochiral/Racemic Biaryls. 19.2.1.3.1. Asymmetric Ring‐Opening Transformation
19.2.1.3.2. Ortho‐C‐H Functionalization
19.2.1.3.3. Functionalization of Ortho‐Substituents
19.2.2. Heterobiaryl Atropisomers. 19.2.2.1. Stereoselective Formation of Aryl‐Heteroaryl Bond
19.2.2.2. De Novo Heteroarene Formation
19.2.2.3. Atroposelective Functionalization of Prochiral/Racemic Heterobiaryls. 19.2.2.3.1. C‐H Functionalization
19.2.2.3.2. Functionalization of Ortho‐Substituent
19.2.2.3.3. Dynamic Kinetic Asymmetric Transformation (DYKAT)
19.2.3. Nonbiaryl Atropisomers. 19.2.3.1. Stereogenic C‐N Axis. 19.2.3.1.1. Stereoselective Formation of C‐N Bond
19.2.3.1.2. Atroposelective Functionalization on Preformed Nonbiaryl C‐N Scaffold
19.2.3.1.3. Atroposelective N‐Annulation
19.2.3.2. Stereogenic C‐C Axis. 19.2.3.2.1. Atroposelective C(aryl)‐C(alkenyl) Bond Formation
19.2.3.2.2. Alkyne Annulation
19.2.3.2.3. Atroposelective Functionalization of Aryl‐Alkene scaffold
19.3. ORGANOCATALYSIS. 19.3.1. Biaryl Atropisomers. 19.3.1.1. Formation of C(aryl)‐C(aryl) Bond
19.3.1.2. De Novo Arene Formation
19.3.1.3. Atroposelective Functionalization of Prochiral/Racemic Biaryls. 19.3.1.3.1. DKR
19.3.1.3.2. Desymmetrization
19.3.1.3.3. KR
19.3.2. Heterobiaryl Atropisomers. 19.3.2.1. Stereoselective Formation of Aryl‐Heteroaryl Bond
19.3.2.2. De Novo Arene Formation. 19.3.2.2.1. N‐Heteroatropisomers
19.3.2.2.2. O‐Heteroatropisomers
19.3.2.3. Atroposelective Functionalization of Prochiral/Racemic Heterobiaryls
19.3.3. Nonbiaryl Atropisomers. 19.3.3.1. Stereogenic C‐N Axis. 19.3.3.1.1. Stereoselective Formation of C‐N Bond
19.3.3.1.2. Atroposelective N‐Annulation
19.3.3.1.3. Atroposelective Functionalization on Preformed Nonbiaryl C‐N Scaffold
19.3.3.2. Stereogenic C‐C Axis: Aromatic Amides. 19.3.3.2.1. De Novo Arene Formation
19.3.3.2.2. Atroposelective Functionalization of Aromatic Amides
19.3.3.3. Stereogenic C‐C Axis: Aryl Alkenes. 19.3.3.3.1. Atroposelective C(aryl)‐C(alkenyl) Bond Formation
19.3.3.3.2. Alkyne Annulation
19.3.3.3.3. Addition reaction of alkynes
19.3.3.3.4. Atroposelective Functionalization of Aryl‐Alkene Scaffold
19.4. ENZYMATIC CATALYSIS. 19.4.1. Biaryl Atropisomers
19.4.2. Heteorobiaryl Atropisomers
19.5. CONCLUSION
REFERENCES
20 ASYMMETRIC SYNTHESIS OF PLANAR CHIRAL AND HELICALLY CHIRAL COMPOUNDS
20.1. INTRODUCTION
20.2. ENANTIOSELECTIVE SYNTHESIS OF PLANAR CHIRAL COMPOUNDS
20.2.1. Enantioselective Synthesis of Planar Chiral Ferrocenes. 20.2.1.1. Pioneering Works
20.2.1.2. Pd‐Catalyzed Intermolecular Reactions Using Amino Acid‐Derived Ligands
20.2.1.3. Intermolecular Reactions Using Other Metal Catalysts
20.2.1.4. Pd‐Catalyzed Intramolecular Cross‐Coupling
20.2.1.5. Rh‐ and Ni‐Catalyzed Dehydrogenative Couplings
20.2.1.6. Au‐ and Pt‐Catalyzed Cycloisomerizations
20.2.2. Enantioselective Synthesis of Planar Chiral Arene‐Chromium Complex
20.2.3. Enantioselective Synthesis of Planar Chiral Cyclophane. 20.2.3.1. Rh‐Catalyzed [2+2+2] Cycloaddition
20.2.3.2. Other Protocols: Coupling and Ortho‐Lithiation
20.2.4. Enantioselective Synthesis of Planar Chiral Cyclic trans‐Alkenes
20.3. ENANTIOSELECTIVE SYNTHESIS OF HELICALLY CHIRAL COMPOUNDS
20.3.1. [2+2+2] Cycloaddition of Alkynes. 20.3.1.1. Enantioselective Synthesis of Carbohelicene Derivatives
20.3.1.2. Enantioselective Synthesis of Heterohelicene Derivatives
20.3.2. Au(I)‐Catalyzed Cycloisomerization of Arylalkynes
20.3.3. Other Protocols
20.4. SUMMARY
REFERENCES
21 ASYMMETRIC POLYMERIZATION
21.1 INTRODUCTION
21.2 ENANTIOSELECTIVE POLYMERIZATION. 21.2.1 Preparation of Optically Active Polyolefins. 21.2.1.1 Polymerization of Vinyl Olefins
21.2.1.2 Polymerization of Diolefins
21.2.1.3 Polymerization of Cyclic Olefins
21.2.2 Preparation of Chiral Polyketones from the Copolymerization of Olefins and CO
21.2.3. Enantioselective Epoxide Homopolymerizations and Copolymerizations
21.2.3.1 Preparation of Chiral Polyethers via Enantioselective Epoxide Homopolymerization
21.2.3.2 Preparation of Chiral Polycarbonates
21.2.3.2.1. Kinetic Resolution Copolymerization of racemic Epoxides with CO2
21.2.3.2.2. Asymmetric Polymerization of Meso‐Epoxides with CO2
21.2.3.3 Preparation of Chiral Poly(monothiocarbonate)s
21.2.3.4 Preparation of Chiral Polyesters
21.2.3.4.1. Enantioselective Polymerization of Lactides and Lactones
21.2.3.4.2. Asymmetric Copolymerization of Epoxides and Cyclic Anhydrides
21.2.4 Other Asymmetric Polymerization Reactions. 21.2.4.1 Asymmetric Condensation Polymerization
21.2.4.2 Oxidative‐Coupling Polymerization
21.3 HELIX‐SENSE‐SELECTIVE POLYMERIZATIONS
21.4 SUMMARY AND OUTLOOK
REFERENCES
22 CONTINUOUS‐FLOW CHEMISTRY IN CATALYTIC ASYMMETRIC SYNTHESIS
22.1. INTRODUCTION
22.2. OVERVIEW OF CATALYTIC PROCESSES IN FLOW ORGANIC SYNTHESIS
22.3. ENANTIOSELECTIVE C–C BOND‐FORMING REACTIONS THROUGH 1,4‐ADDITION REACTIONS
22.4. ENANTIOSELECTIVE C–C BOND‐FORMING REACTIONS THROUGH 1,2‐ADDITION REACTIONS
22.5. ENANTIOSELECTIVE C–C BOND FORMATION THROUGH CYCLOADDITION REACTIONS
22.6. ENANTIOSELECTIVE C–C BOND‐FORMING REACTIONS THROUGH HYDROFORMYLATION
22.7. ENANTIOSELECTIVE C–X BOND‐FORMING REACTIONS THROUGH OXIDATIVE PROCESSES
22.8. ENANTIOSELECTIVE REDUCTION OF DOUBLE BONDS THROUGH CONTINUOUS‐FLOW SYSTEMS
22.9. CONCLUSION AND OUTLOOK
REFERENCES
INDEX
WILEY END USER LICENSE AGREEMENT
Отрывок из книги
Fourth Edition
.....
Schneider reported a domino‐type reaction between diazoesters and ortho‐quinone methides generated in situ to furnish densely functionalized chromans with three contiguous stereogenic centers. A transition metal and a Brønsted acid catalyst 6b acted synergistically to produce a transient oxonium ylide and ortho‐quinone methide, which underwent subsequent coupling in a conjugate‐addition‐hemiacetalization event to afford chromans (Scheme 2.47) [99].
Scheme 2.47. Synergistic rhodium/phosphoric acid catalysis.
.....