Electron Transfer
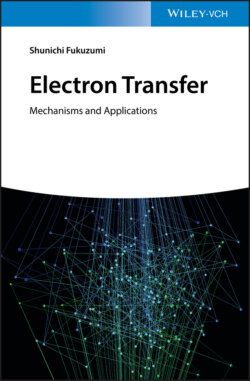
Реклама. ООО «ЛитРес», ИНН: 7719571260.
Оглавление
Shunichi Fukuzumi. Electron Transfer
Table of Contents
List of Illustrations
Guide
Pages
Electron Transfer. Mechanisms and Applications
Copyright
Acknowledgments
1 Introduction
2 Marcus Theory of Electron Transfer
3 Photosynthetic Reaction Center Models
4 Electron Donor–Acceptor Dyads
5 Supramolecular Electron Transfer
5.1 Cation–Anion Binding
5.2 π‐Complexes
5.3 Electron‐Transfer Switching
5.4 Dendrimers
5.5 Supramolecular Solar Cells
6 Effects of Metal Ions on Photoinduced Electron Transfer
7 Photoredox Catalysis. 7.1 Photocatalytic Oxygenation
7.2 Photocatalytic Oxibromination
7.3 CarbonCarbon Bond Formation
7.4 DNA Cleavage
7.5 Anti‐Markovnikov Hydroetherification
7.6 Photocatalytic Cycloaddition
7.7 Photocatalytic Hydrotrifluoromethylation
7.8 Photocatalytic Hydrogen Evolution
8 Hydrogen Storage
8.1 Interconversion Between Hydrogen and Formic Acid
8.2 Interconversion Between Hydrogen and NADH
8.3 Hydrogen Evolution from Alcohols
8.4 Hydrogen Evolution from Paraformaldehyde
9 Metal Ion‐Coupled Electron Transfer (MCET) 9.1 MCET of O2
9.2 Binding Modes of Metal Ions
9.3 Self‐Organized MCET
9.4 Accelerating and Decelerating Effects of Metal Ions
9.5 Driving Force Dependence of MCET Rate Constants
9.6 MCET Coupled with Hydrogen Bonding
9.7 MCET Catalysis. 9.7.1 Hydride Transfer vs. Cycloaddition
9.7.2 Suproxode Disumutase (SOD) Models
9.8 MCET of Metal‐Oxo Complexes
9.9 PCET of Metal‐Oxo Complexes
9.10 Unified Mechanism of MCET and PCET of Metal‐Oxo Complexes
9.11 MCET of Metal‐Peroxo Complexes
10 Catalytic Reduction of O2
11 Catalytic Oxidation of H2O
12 Production of Hydrogen Peroxide from Water and Oxygen as a Solar Fuel
13 Production and Usage of Hydrogen Peroxide as a Solar Fuel in Seawater
14 Photosystem II Mimic
15 Conclusion and Perspective
References
Index
WILEY END USER LICENSE AGREEMENT
Отрывок из книги
Shunichi Fukuzumi
Electron transfer is the most fundamental chemical reaction in which only electron is removed or attached. However, it is quite important to recognize the fundamental difference of electron‐transfer reactions from other chemical reactions in which chemical bonds are cleaved and formed during the reactions. Because electron transfer occurs based on the Franck–Condon principle, nuclear configurations remain the same before and after the electron transfer [6]. In contrast, nuclear configurations are changed significantly in chemical reactions associated with the cleavage and formation of chemical bonds. The fastest electron transfer is achieved with no activation energy when the nuclear configurations are the same before and after the electron transfer with a large driving force of electron transfer. When the driving force of electron transfer is further increased, the nuclear configurations are not the same any more before and after the electron transfer. In such a case, activation energy is again required to change the nuclear configurations before the electron transfer to be the same as that after the electron transfer. This means that the rate of electron transfer is slowed down with increase in the driving force of electron transfer. This region is called the Marcus inverted region, which is discussed in detail later [6]. The importance of the Marcus inverted region is well recognized in the function of the photosynthetic reaction center (vide infra) [7–9].
.....
In contrast to the photoirradiation of a purified PhCN solution of Acr+–Mes at 298 K, which results in no change in the absorption spectrum (Figure 4.4a), when the photoirradiation of the same solution was performed at low temperatures (213–243 K) with a 1000 W high‐pressure mercury lamp through the UV light cutting filter (>390 nm) and the sample was cooled to 77 K, the color of the frozen sample at 77 K was clearly changed as shown in the inset of Figure 4.4b. When a glassy 2‐methyltetrahydrofuran (2‐MeTHF) is employed for the photoirradiation of Acr+–Mes at low temperature, the resulting glassy solution measured at 77 K affords the absorption spectrum due to the electron‐transfer state, which consists of the absorption bands of the Acr· moiety (500 nm) and the Mes·+ moiety (470 nm) as shown in Figure 4.4b. No decay of the absorption due to the electron‐transfer state in Figure 4.2b was observed until liquid nitrogen ran out [65].
The long lifetime of the ET state of Acr+–Mes has allowed observing the structural change in the Acr+–Mes(ClO4−) crystal upon photoinduced ET directly by using laser pump and X‐ray probe crystallographic analysis (Figure 4.5) [72]. Upon photoexcitation of the crystal of Acr+–Mes(ClO4−), the N‐methyl group of the Acr+ moiety was bent and its bending angle was 10.3(16)° when the N‐methyl carbon moved 0.27(4) Å away from the mean plane of the ring as shown in Figure 4.5 [72]. This bending is caused by the photoinduced electron transfer from the Mes moiety to the Acr+ moiety to produce Acr·–Mes·+, because the sp2 carbon of the N‐methyl group of Acr+ is changed to the sp3 carbon in the one‐electron reduced state (Acr·) [72]. The bending of the N‐methyl group by photoexcitation was accompanied by the rotation and movement of the ClO4− by the electrostatic interaction with the Mes·+ moiety (Figure 4.5) [72]. Thus, the observed bending of the N‐methyl group and the movement of ClO4− provide strong evidence for the generation of the ET state of Acr+–Mes upon photoexcitation. In contrast to the case of Acr+–Mes, no geometrical difference was observed upon photoexcitation of Acr+–Ph, which does not afford the ET state [72].
.....